Zeroing in on the path length dependence of jet quenching: High pT azimuthal anisotropy of π0 in Au+Au Collisions at RHIC
October 12, 2010
By Jiangyong Jia, Assistant Professor of Chemistry at Stony Brook University and a member of the PHENIX Collaboration.
A common tool used to study the properties of the strongly interacting Quark Gluon Plasma (sQGP) is the azimuthal anisotropy of particles emitted in the transverse plane. A non-zero anisotropy can be traced to the elliptic shape of the initial fireball and the strong final state interaction which transfers such spacial asymmetry into the transverse momentum (pT) space. This anisotropy probes the interactions of the sQGP, which can be understood in terms of two different phenomena depending on the pT. For low pT hadrons, the azimuthal anisotropy is driven by the anisotropic collective expansion (or flow) as a result of the anisotropic pressure gradient built up in the collision zone. For high pT hadrons, it reflects the path length (l) dependence of energy loss. That is, jet yields are more suppressed along the long axis of the fireball than its short axis. As a result, the strength of the anisotropy at RHIC exhibits a characteristic pT dependence: it first increases with pT (reflecting the collective expansion), then drops with pT (reflecting the impediment of flow due to viscosity), and remains positive at higher pT (reflecting the effects of jet quenching).
Experimentally, the azimuthal anisotropy is quantified via a Fourier analysis of the distribution of the azimuthal emission angle ∆φ of particles relative to the reaction plane (RP): Due to the almost elliptic shape of the initial geometry, the anisotropy is dominated by the second harmonic term: [(d2N)/(dpTd∆φ)]  ∼ 1+2v2(pT)cos(2∆φ), where v2(pT) characterizes the strength of the anisotropy as a function of pT. The precision of the measurement depends on the magnitude of the raw signal v2raw, which in turn is related to the true v2 value and the RP resolution factor σRP: v2raw = v2 σRP, where σRP=〈cos2∆Ψ〉 accounts for dilution due to the dispersion ∆Ψ of the RP angle. Note that it is always smaller than unity.
For Au+Au collisions at top RHIC energy, it is well known that the low pT v2 data can be described by relativistic viscous hydrodynamics models. In contrast, the high pT v2 data from early RHIC measurements exceeded calculations from jet quenching models based on perturbative QCD (pQCD) framework [1]. However, these measurements had rather limited pT reach ( <~ 6 GeV/c), thus they could be influenced by collective flow and recombination effects, rather than jet quenching. Furthermore, it was unclear how a possible bias to the RP determination, from di-jets, influenced the measured v2 values. The essential point here is that, meaningful comparisons between data and theory require precision v2 data, as well as a good understanding of influence of a possible jet bias.
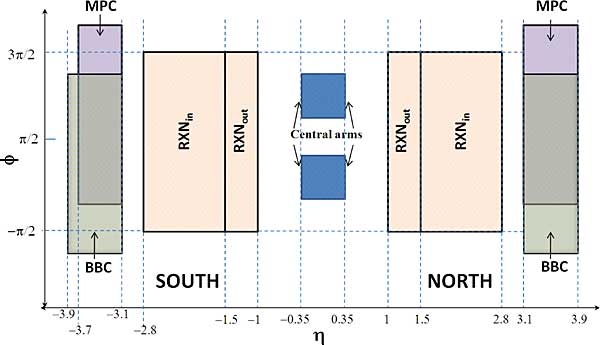
Figure 1: The figure shows the azimuthal angle (φ) and pseudo-rapidity (η) acceptance of PHENIX detectors used for reaction plane (RP) measurement, together with the central arm acceptances for π0 measurement. All RP detectors have full coverage in azimuth. The BBC and MPC are shifted vertically relative to each other to improve the visibility; The table summarize, for each detector, the particles detected and the RP resolution factor σRP for 25%-30% centrality selection, where it reaches maximum. Note that v2 is obtained from raw signal as v2=v2raw/σRP, thus larger σRP implies smaller correction, hence more precision.
Detectors | Capability | maximum resolution |
σRP | ||
BBC | h± | 0.41 |
MPC | h±,γ, | 0.53 |
neutral hadrons | ||
RXNin | h±,γ | 0.65 |
RXNout | h±,γ | 0.62 |
MPC+RXNin | - | 0.73 |
In a recent publication "Azimuthal anisotropy of π0 production in Au+Au collisions at √{sNN}=200 GeV: Path-length dependence of jet-quenching and the role of initial geometry" [2], PHENIX addressed both limitations via new measurements using RUN7 data, which incorporated several forward detectors covering a broad rapidity ranges (shown in Figure 1). These detectors not only provided more precise RP measurements, but also allowed systematic assessment of the jet bias. We do the latter by varying the rapidity gap between the π0 measured in the PHENIX central arms (η < 0.35) and the RP. We find that the v2 measured with the RPs from MPC and RXNin (see Fig. 1) are very consistent, indicating that they were not influenced by jet bias. Thus, the signal from these two detectors were combined to provide a single RP measurement. The large dataset and improved RP resolution from RUN7 gave an equivalent of  ∼ 15 fold increase in statistics over our previous measurement of π0 v2 from RUN4.
Figure 2 (a)-(c) shows v2(pT) spanning 1-18 GeV/c for three centrality bins. The v2 values above 3 GeV/c indicate a slow decrease up to 7-10 GeV/c, and then remain significantly above zero at higher pT. The ratios in Fig. 2 (d)-(f) confirm the consistency of v2 measured using the RP from the MPC or the RXNin and imply that the influence of rapidity dependent jet bias is within the uncertainty of the measurement.
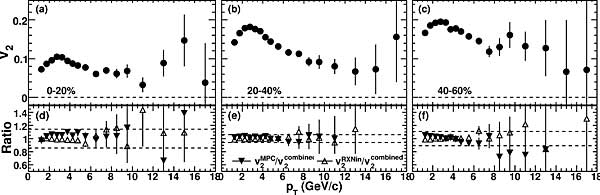
Figure 2: (a)-(c): π0 v2 using combined reaction plane for MPC and RXNin as a function of pT in 20% centrality bins (they are combined from the 10% centrality bin results in the paper). (d)-(f): ratios of v2 measured separately using MPC (solid triangles) and RXNin (open triangles) to combined result; the dashed lines indicate the systematic error. Note that MPC and RXNin are combined at raw hit level before the RP flattening correlatio which unfolds for non-uniform detector acceptance; thus combined v2 is not a simple weighted average of v2MPC and v2RXNin.
The precision v2 data at high pT provide valuable insights to our current understanding of the jet quenching picture. A good way to present the data is to plot the centrality dependence of the v2 in small bins, but integrated over a broad pT range to reduce the error bar. This is done in Figure 3 (a)-(b) for two high pT selections. They are compared to various pQCD model calculations. These models are calculated with different geometry and different implementation of energy loss processes. For example, the HT and ASW models include only coherent radiative energy loss, while the AMY and WHDG models also include collisional energy loss. All these models significantly under-predict the v2 data.
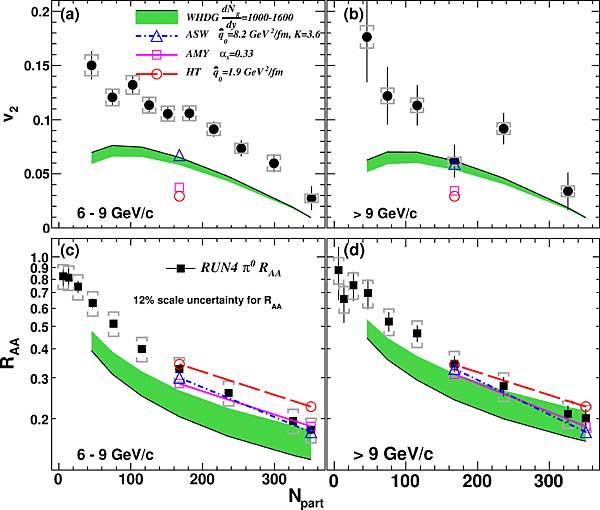
Figure 3: (a)-(b) v2 vs Npart in two pT ranges; (c)-(d) RAA vs Npart in same pT ranges. Each are compared with four pQCD models from [3] (AMY, HT, ASW) with parameters quoted for quark jet at τ0=0.6 fm/c, and [4](WHDG). Log-scale is used for RAA to better visualize model calculations. Note that the gluon density [(dNg)/dy]=1000 of WHDG corresponds to lower (upper) boundary of the shaded bands for v2 (RAA), while [(dNg)/dy]=1600 corresponds to upper (lower) boundary for v2 (RAA).
In current jet quenching pictures, inclusive suppression RAA and v2 are anti-correlated, i.e. a small RAA implies a large v2 and vice versa. Consequently, more information can be obtained by comparing the data with a given model for both RAA and v2. Fig. 3 (c)-(d) compares the centrality dependence of π0 RAA data to four model calculations for the same pT ranges. The level of agreement with RAA is not perfect (for example, WHDG systematically under-predicts the data toward the peripheral collisions), but the deviation from the data is certainly less dramatic than that for the v2(Npart). This, together with the large spread among the calculations, indicate that v2 should be very useful to understand the deficiencies in current pQCD models.
How to resolve this apparent discrepancies between data and theories? Most jet quenching calculations assume a smooth Woods-Saxon nuclear geometry. Such geometry ignores event-by-event shape distortions due to spatial fluctuations of participating nucleons, and a possible overall shape distortion due to gluon saturation effects, known as the CGC geometry. A recent estimation [5] shows that the calculated v2 could be increased by 30-40% when including these effects, however, it still falls below the data. Unless there are additional modifications to the collision geometry that we are not aware of, this large discrepancy likely implies that our current understanding of the pQCD-based energy loss is not complete. For example, the quadratic l dependence formula for radiative energy loss [6],
|
may need to be modified to increase the anisotropy. There are three ideas along this line of reasoning. Liao and Shuryak [7] do this by requiring that most energy loss in sQGP is concentrated around Tc; such a non-monotonic dependence of energy loss with energy density achieves good agreement with the data. Alternatively, one may reproduce the data by increasing the formation time τ0 in Eq. 1 from default value of < 0.6 fm/c to 1.5−2.0 fm/c [8,5]. However, this is inconsistent with the hydro calculation which requires interaction at the early stage of the evolution. Finally, one can increase the v2 by modifying the l dependence of Eq. 1 to a cubic dependence
|
This cubic dependence is expected in certain non-perturbative energy loss calculations based on AdS/CFT gravity-gauge dual theory [9]. Indeed, our data agrees very well with one such calculation [10] (see original paper for details).
Admittedly, the model implementations of these ideas are still rather qualitative in nature. Whether they are correct or not requires more rigorous theoretical developments, with a similar level of sophistication as implemented in the pQCD based approaches. Nevertheless, our precision data have already begun to provide key constraints on the initial geometry, medium space-time evolution, and the jet quenching mechanisms.
References
- [1]
- E. V. Shuryak, Phys. Rev. C 66, 027902 (2002)
- [2]
- A. Adare et al. [PHENIX Collaboration], arXiv:1006.3740 [nucl-ex], accepted by PRL.
- [3]
- S. A. Bass et al. Phys. Rev. C 79, 024901 (2009)
- [4]
- S. Wicks, W. Horowitz, M. Djordjevic and M. Gyulassy, Nucl. Phys. A 784, 426 (2007);
- [5]
- J. Jia and R. Wei, Phys. Rev. C 82, 024902 (2010)
- [6]
- H. Zhang, J. F. Owens, E. Wang and X. N. Wang, Phys. Rev. Lett. 98, 212301 (2007)
- [7]
- J. Liao and E. Shuryak, Phys. Rev. Lett. 102, 202302 (2009)
- [8]
- V. S. Pantuev, JETP Lett. 85, 104 (2007)
- [9]
- F. Dominguez, C. Marquet, A. H. Mueller, B. Wu and B. W. Xiao, Nucl. Phys. A 811, 197 (2008)
- [10]
- C. Marquet and T. Renk, Phys. Lett. B 685, 270 (2010)
2010-2063 | INT/EXT | Newsroom