CFN User Spotlight: Morgan Huse Measures the Mechanical Forces Generated by Immune Cells
interview with a CFN user
January 25, 2019
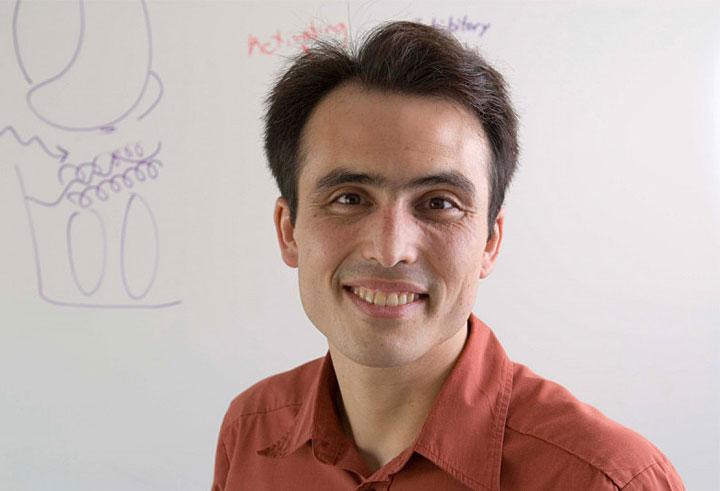
Immunologist Morgan Huse runs a lab at Memorial Sloan Kettering Cancer Center that focuses on the study of immune cell communication. Source: Memorial Sloan Kettering Cancer Center.
How do immune cells communicate? Immunologist Morgan Huse of Sloan Kettering Institute’s Immunology Program—part of Memorial Sloan Kettering Cancer Center—is trying to answer this very question. His studies of the structure and function of immune cell-cell interactions have brought him to the Center for Functional Nanomaterials (CFN), a U.S. Department of Energy (DOE) Office of Science User Facility at Brookhaven National Laboratory. Here, he uses microfabrication tools and electron microscopes to generate micropatterned elastic surfaces composed of flexible micropillars. He then uses these surfaces to measure the mechanical forces exerted by T cells, a type of white blood cell that mediates immunity against viruses and incipient tumors. A better understanding of how T cells interact with and destroy infected or cancerous target cells could provide the foundation for harnessing the immune system to treat cancer and other diseases. Huse holds a PhD in structural biology from Rockefeller University and a bachelor’s degree in biochemistry from Harvard University. He joined Memorial Sloan Kettering in 2007, after completing a postdoc at Stanford University.
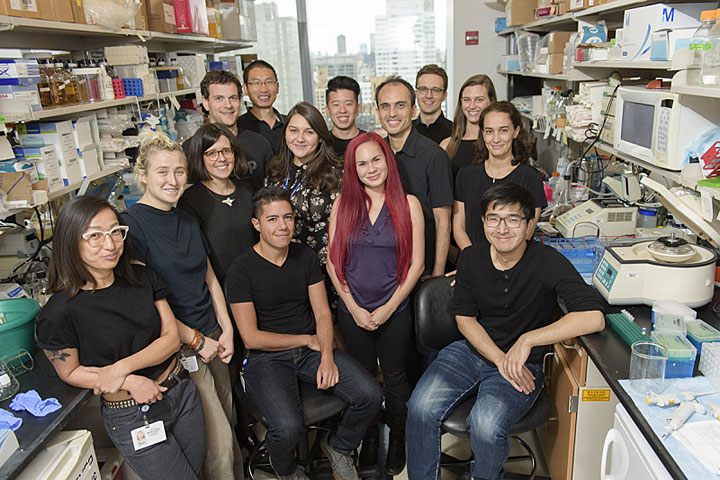
The Morgan Huse Lab consists of postdocs and students with backgrounds in immunology, cell biology, and biophysics. Source: Memorial Sloan Kettering Cancer Center.
Memorial Sloan Kettering Cancer Center is known around the world as a leading cancer treatment and research facility. How does your research play into its mission?
My lab is part of the Immunology Program at Memorial Sloan Kettering. Broadly speaking, research conducted within this program focuses on understanding immune mechanisms that could allow us to better use the immune system to kill tumors. In my lab specifically, we study the intercellular contacts formed by immune cells, which mediate a huge fraction of their intercellular communication by forming transient but structurally specific interactions with other cells.
Immune cells are remarkable in that they retain many of the properties of developing cells, but in the context of an adult animal. They can change their shape quickly and dramatically, undergo extensive migration into a variety of tissues, and of course form structurally specific, communicative cell-cell contacts. In general, the structure of each of these contacts is thought to enhance the potency and specificity of their respective functions.
Recently, we have been focusing on the interactions between cytotoxic T lymphocytes—a type of white blood cell—and the infected or cancerous cells they kill. Killing is essentially intercellular communication that instructs the target cell to die. Cytotoxic lymphocytes do this by forming a contact with the target cell and secreting “venomous” proteins into the interface between the two cells. These proteins destroy the target cell.
It turns out that there is an important physical component to the interaction. The T cell actually grabs onto the target cell, deforming its surface. It is a very dynamic interaction that transmits a lot of mechanical force. In the last few years, my lab has been trying to measure these mechanical forces and understand how they contribute to the killing process.
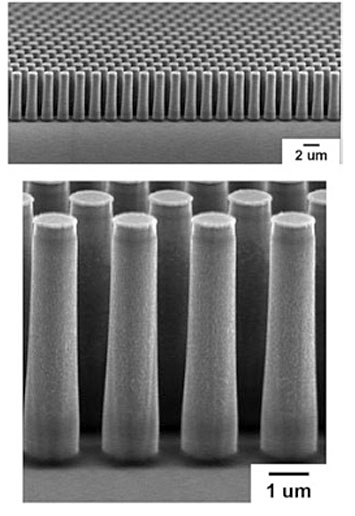
Scanning electron microscope images of micropillar arrays at low (top) and high (bottom) magnification. Source: The Immune System Response: Methods and Protocols, Chapter 19: "Probing Synaptic Biomechanics Using Micropillar Arrays," pp. 333–346.
How do you study the biomechanics of immune cell-cell interactions?
Together with Lance Kam, who directs the Microscale Biocomplexity Laboratory in the Department of Biomedical Engineering at Columbia University, we generate arrays of micropillars that are made out of polydimethylsiloxane (PDMS), which is a kind of deformable plastic. These micropillars are very small; the ones we use for force measurements are 0.7 microns in diameter and six microns tall, and we place them in arrays where they are two microns apart center to center.
We coat the micropillar arrays with proteins so that they look like target cells that T cells would want to kill. Then, we throw T cells at the arrays. Because the pillars are deformable, when the T cells grab onto the arrays, they bend the micropillars. Each of these micropillar deflections correspond to a force vector. We can look at the distribution of forces transmitted by the T cells in space, using imaging experiments to correlate the force exertion with other processes occurring within the cell—for example, the secretion of venomous proteins or the accumulation of a cytoskeletal molecule in a certain location.
One current working hypothesis, which is based on some other experiments we have carried out in the lab, is that mechanical force contributes to killing by straining the surface of the target cell and making it easier for perforin—a type of venomous protein—to form pores in the membrane of the target cell. It’s kind of like when you’re trying to pop a partially deflated balloon with a needle, it helps if you squeeze the balloon first. We think that T cells are doing a similar sort of thing.
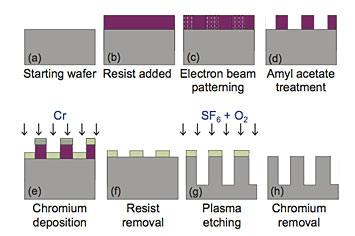
A schematic of the process used to fabricate silicon masters. The silicon wafer is shown in gray, the electron-beam resist in purple, and chromium in green. Chromium is used to create a physical mask to etch pillars into the silicon substrate and is removed at a later step in the process. Source: The Immune System Response: Methods and Protocols, Chapter 19: "Probing Synaptic Biomechanics Using Micropillar Arrays," pp. 333–346.
How do you generate the micropillar arrays?
First, we use the microfabrication technique of electron-beam lithography to etch a hexagonal micropillar array onto a wafer of silicon. This version is called the silicon “master.” Then, we pour the deformable plastic (PDMS) onto the master and cure it. When we peel the hardened plastic off, we are left with a mold that contains an inverse shape of the pillars—holes. We then stamp this negative mold onto glass to cast a positive version of the PDMS micropillar array that the T cells can interact with. Once the pillars are cured so that they stick to the glass, we coat them with proteins and fluorescent chemicals called fluorophores and add T cells.
When we started collaborating with the Kam Lab at Columbia, we were using old silicon masters that had been sitting around for some years. The facility that his lab had used to generate the masters was no longer available, so we needed to find a new place to do this microfabrication work. Quite fortuitously, I met CFN Director Chuck Black at a party a few years ago, as a result of our daughters attending the same school. He told me about an amazing facility at Brookhaven, the CFN.
Since then, my lab members have been coming to the CFN to make the silicon masters. Chuck has actually made several silicon masters for us himself, as well.
The Nanofabrication Facility at the CFN has a suite of equipment for making nano- and micron-sized materials and devices. Which instruments does your lab use at the CFN to fabricate the silicon masters?
We use the electron-beam lithography tool, chlorine-chemistry etcher, and thin-film deposition system. We also use a scanning electron microscope (SEM) at the CFN to take pictures of the masters once they have been made.
One of the disadvantages of working at a hospital is that there is no engineering department, so we are quite lacking in our microfabrication capabilities. The instruments at the CFN are state of the art.
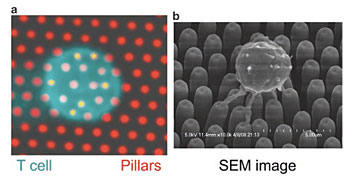
(a) A fluorescence image of a cytotoxic T cell bound to micropillars coated with stimulatory proteins. The T cell is teal-colored, and the pillars are red. Yellow asterisks indicate substantial pillar deflections. (b) Scanning electron microscope image of a T cell bound to micropillars. Source: The Immune System Response: Methods and Protocols, Chapter 19: "Probing Synaptic Biomechanics Using Micropillar Arrays," pp. 333–346.
How do you monitor the interactions between the T cells and pillars, and what have you discovered in monitoring these interactions?
We use various microscopy tools in our lab and in the imaging facility at Sloan Kettering. In particular, we use fluorescence microscopy. The fluorophores we introduce into the sample help us visualize what is happening. They are excited by light of a certain wavelength and emit light of a longer wavelength. We collect this emitted light, or fluorescence, on a camera. Typically, we record images every 15 seconds for 30 minutes.
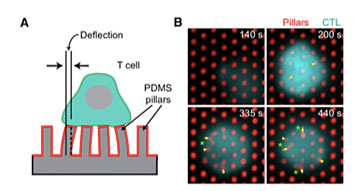
(A) A schematic of the micropillar system. When the T cell comes into contact with the micropillars expressing certain molecules, it bends them. These deflections are captured over time (B). Large pillar deflections are indicated in yellow, and hotspots of strong force exertion are indicated in green. Source: Cell 165, 100–110, March 24, 2016.
Using automated software, we then track the positions of the pillars over time to determine the deflections. The mechanical force of each deflection can be calculated based on the dimensions and composition of the pillars and the deflection length.
From our studies, we have discovered that T cells do kill to a certain extent like venomous snakes. They kill with venom but couple that with a physical delivery system that makes the venom more powerful, similar to a snake bite. In other words, the physical force exerted by T cells at the T cell-target cell interface makes the killing of the target cell more efficient.
Immunotherapy—a form of therapy in which a patient’s own immune system is used to fight disease—is currently a hot topic in medicine because of its promise in treating cancer, as well as autoimmune conditions. How does your lab’s research on T cells relate to immunotherapy?
Cytotoxicity is generally thought to play a significant part in T cell function both in anticancer immunotherapy and in autoimmunity. It is out hope that by better understanding how T cells kill, we can better modulate their activity in therapeutic settings.
What is needed to make further advances in your field?
We need tools that will allow us to measure forces not only more accurately but also in contexts that are more physiologically relevant. Right now, we can measure cellular forces in very controlled, artificial environments, but T cells do not live on hexagonal arrays of plastic micropillars. We need the ability to measure mechanical output in the environments where T cells and other immune cells actually live.
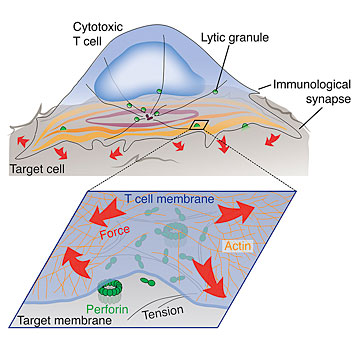
The immunological synapse formed between a cytotoxic T cell and an infected or a transformed target cell is a physically active structure capable of exerting mechanical force. The force exerted across this synapse drives the killing of the target cell by enhancing the activity of a protein called perforin. Source: Cell 165, 100–110, March 24, 2016.
In order to measure mechanical changes, you really need mechanical probes—ones that will behave in predictable, calibratable ways—but living things are not good mechanical probes. It is very challenging to make small mechanical probes that are highly sensitive and can be put into different contexts. For example, we have hypothesized that the mechanical forces exerted by T cells on the surface of a target cell induce changes in local membrane tension on that surface. However, we have not been able to rigorously test this hypothesis because we do not have a good way of monitoring local membrane tension. It would be fantastic if we had some sort of fluorescent probe in the target cell that would tell us where the cell is experiencing stress and strain.
A better understanding of these processes will hopefully present avenues for regulating the immune system. One of the current issues with immunotherapy—and this is an oversimplified explanation—is that we are really good at turning everything on and turning everything off, but when we turn everything on, we get a fair amount of bad along with the good. In other words, your immune cells will attack the tumor cells but they will also attack normal healthy tissue. Achieving specificity is a formidable challenge.
What excites you most about your research and the field of immunotherapy?
I suppose the idea that immune cells have this really complex mechanical life—that their movement and their shape changes have meaning. That’s very exciting to me.
One of the promises of immunotherapy is that it is adaptable in the sense that if you elicit an immune response in the right way, the immune system will adapt to face the specific challenge present in a patient. Essentially, the immune system will attack tumors in a multifactorial way, unlike small molecule-based therapies that target one molecule in one way. Those sorts of therapies may be effective in the short term but are prone to resistance mutations. If you target cancer with one agent, the cancer can mutate and that will drive relapse. An immune system that attacks in a broader multifactorial way is more robust, giving you “multidrug” therapy in a personalized manner.
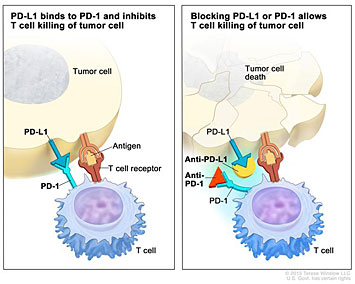
Checkpoint proteins—such as PD-L1 on tumor cells and PD-1 on T cells—help keep immune responses in check. The binding of PD-L1 to PD-1 keeps T cells from killing tumor cells (left). Using an immune checkpoint inhibitor (anti-PD-L1 or anti-PD-1) to block this binding allows the T cells to kill these tumor cells (right). Source: National Cancer Institute.
How do you see immunotherapy advancing with respect to more established cancer treatments, such as chemotherapy and radiation?
Marrying immunotherapy with more established approaches is a very active area of research. For example, there are indications that you can actually stimulate an immune response in cancer patients by creating damage in a tumor through radiation therapy. The radiation induces the release of molecules that activate the immune system.
You may have heard of immune checkpoint blockade therapy; it has been getting a lot of attention lately with regard to melanoma and non-small-cell lung cancer. Checkpoints are proteins that regulate immune system activity. In certain therapeutic contexts, they must be overcome for a productive immune response to occur. Cancer cells can sometimes evade immune system attacks by switching T cells to an inactive (off) state. In checkpoint blockade therapy, patients get antibody drugs that relieve these inhibitory pathways. Thus, the T cells that have been switched off can come back to “life” to attack a tumor.
This therapy works best when there are enough tumor antigens, which are molecules that the immune system can use to recognize tumors. It turns out that the number of antigens is very much correlated with the number of mutations present in a tumor. If a tumor has lots of mutations, it makes more antigens. In turn, the immune system can more easily detect the tumor. Melanoma and non-small-cell lung cancer both have a high level of mutation.
However, one of the deleterious side effects of checkpoint blockade immunotherapy is that it can lead to autoimmune-related events, such as those involving the skin and the gut.
Your research is on the cutting edge of this exciting new field. How did you get into immunology, and what does your general interest in science stem from?
I have liked science ever since I was a teenager. In school, I was drawn to science rather than other subjects because I like the prospect of knowing things precisely. I also like knowing things before other people know them.
I got into immunology by chance. I studied structural biology for my PhD and then headed to Stanford for my postdoc. Initially, I was in a neuroscience lab but that did not work out for various reasons, so I had to find another lab. I wanted to stay at the same institution because my wife was there, and I ended up in Mark Davis’ lab, which studied T cells and immune cell-cell interactions. I’ve always been interested in how complex molecular signaling assemblies achieve complex results at a cellular level; the immune system is an incredibly rich source of research projects with precisely these properties.
Brookhaven National Laboratory is supported by the Office of Science of the U.S. Department of Energy. The Office of Science is the single largest supporter of basic research in the physical sciences in the United States, and is working to address some of the most pressing challenges of our time. For more information, please visit science.energy.gov.
Follow @BrookhavenLab on Twitter or find us on Facebook.
2019-14360 | INT/EXT | Newsroom