The Glue that Binds Us All
How an electron-ion collider could help unravel what makes matter stick together and what puts the spin on protons
June 12, 2012
RHIC, the Relativistic Heavy Ion Collider at Brookhaven Lab, found it first: a “perfect” liquid of strongly interacting quarks and gluons — a quark-gluon plasma (QGP) — produced by slamming heavy ions together at close to the speed of light. The fact that the QGP produced in these particle smashups was a liquid and not the expected gas, and that it flowed like a nearly frictionless fluid, took the physics world by surprise. These findings, now confirmed by heavy-ion experiments at the Large Hadron Collider (LHC) in Europe, have raised compelling new questions about the nature of matter and the strong force that holds the visible universe together.
This animation shows the strength of the gluon force field between a quark (at the center of the images) and an anti-quark, which grows as the energy of the nucleus increases. At low boost energies, the force fields are spread out (shown by larger "blobs") and are comparable to the "strong" force that binds quarks together in the proton. At higher boost energies, force field fluctuations are incredibly strong — 10 times greater than the typical strong force — and localized at much shorter distances than the proton size. These "higher resolution" snapshots are cleaner to interpret than those at low boost energies and provide important clues to figuring out the nature of the glue binding together visible matter in the universe.
Similarly, searches for the source of “missing” proton spin at RHIC have opened a deeper mystery: So far, it’s nowhere to be found.
To probe these and other puzzles, nuclear physicists would like to build a new machine: an electron-ion collider (EIC) designed to shine a very bright “light” on both protons and heavy ions to reveal their inner secrets.
“An electron-ion collider would be the brightest, highest-intensity ‘femtoscope’ to shine on the structure of matter,” said Brookhaven theoretical physicist Raju Venugopalan, referring to its ability to discern structures at the scale of femtometers — that’s 10-15 meters, a millionth of a nanometer, or a millionth of a billionth of a meter!
“Snapshots” of matter at that scale over a wide range of energies would offer deeper insight into the substructure of the nucleus, its constituents, and particularly its smallest components, the quarks and gluons and how they interact.
“Increasingly, it’s looking as if gluons and their interactions may hold the keys to many of our puzzles,” Venugopalan said. An electron-ion collider would be the ideal tool for gazing at the “glue” under conditions where scientists believe that it completely dominates the structure of neutrons, protons, and nuclei.
Glue holds the key
The simplest view of a proton or neutron (the most common hadrons, or particles composed of quarks and gluons) reveals three quarks interacting via the exchange of gluons. These gluons are carriers of the strong force, which “glues” the quarks together, similar to the way photons, particles of electromagnetic energy (or light), carry the electromagnetic force that governs interactions among charged particles.
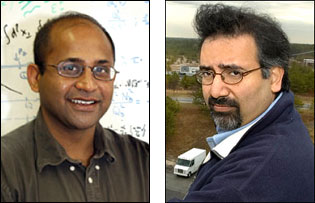
Raju Venugopalan (L) and Abhay Deshpande, two physicists with a strong interest in the physics case for an electron-ion collider.
But the analogy is not quite perfect because, while photons do not interact with one another, gluons do. And experiments probing proton structure at the HERA collider at Germany’s DESY laboratory, along with the increasing body of evidence from RHIC and LHC, suggest that those interactions make all the difference. They result in a much more complex picture of proton structure, with countless gluons (and a sea of quarks and antiquarks) flitting into and out of existence from the vacuum within each hadron.
“Think of the proton as a black night with the gluons like fireflies blinking on and off,” Venugopalan said. “A snapshot with the proton at rest will reveal an ephemeral and diffuse glow from the fireflies whose flicker is barely visible in the darkness. With finer resolution snapshots, one may be able to determine the density of fireflies, localize them better spatially, and even extract details about their motion as they whirl about.”
The experiments at HERA made it clear that getting more details about the gluons is essential because, at high energy, the fleeting gluons become vastly more numerous than the quarks.
“People found this very intriguing, that there were so many gluons, suggesting they play a much bigger role in nuclear structure and interactions than had been previously thought,” he said.
A different spin
Around the same time, physicists looking for the missing source of proton spin — which was clearly shown in fixed-target experiments at CERN, SLAC, and DESY not to be accounted for by the net spin of all the quarks and antiquarks inside — became interested in gluons, too. Could the gluons carry the missing spin? Physicists have been measuring some aspects of gluon spin in collisions between beams of polarized protons at RHIC. But so far, in the energy ranges they’ve explored, the gluon contribution to overall proton spin appears to be near zero. They’ve only looked in regions where the gluons do not yet completely dominate proton structure, however.
“One thing we have not done in spin is explore the contribution of gluons where they exist in large numbers,” said Stony Brook University physicist Abhay Deshpande. To do that, they have to ramp up the energy to look deeper into the proton.
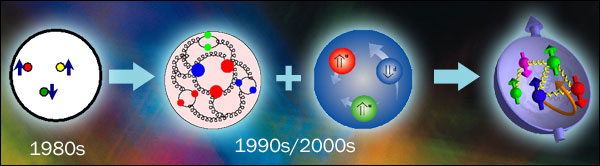
Physicists' understanding of proton spin has evolved from the early view that the spins of the proton's three constituent quarks should make up most if not all the proton's spin (left), to one in which the gluons and the motion of the quarks and gluons — including an orbital component of that motion — can also play significant roles (center). Current and planned investigations of the angular motion of quarks and gluons — the latter to be carried out at a future electron-ion collider (right) — may finally help scientists resolve the mystery of the missing source of spin.
Light speed “magic”
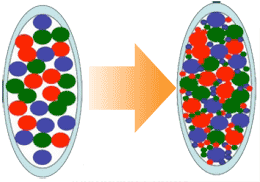
As heavy ions are accelerated to near light speed, fleeting gluons appear to last longer, resulting in rapid growth in the number of gluons and dense, or saturated, gluon force fields. Probing these gluon fields directly with an electron-ion collider would help scientists better understand the strong force they carry to hold together the quarks of ordinary matter, and how they contribute to proton spin.
The closer the particles get to the speed of light, the longer the gluons that arise from the vacuum’s quantum fluctuations last.
“It’s a direct effect of time dilation, part of Einstein’s theory of relativity,” Venugopalan explained. That is, when something is accelerated close to the speed of light, time and the processes that take place inside the particles appear to slow down. “An outside ‘observer’ viewing a fast moving proton would see the glow of the firefly-like gluons last longer and longer the closer the proton got to the speed of light,” he said.
So, in effect, by speeding the particles up, scientists can slow down the gluon fluctuations popping in and out of the vacuum to “take pictures” of them with their particle detectors.
Physicists like Deshpande and others interested in proton spin would like to look at the long-lasting gluons to tease apart their contributions to spin — including how their own orbital motion affects this intrinsic property.
“All the experiments that have looked at proton spin so far have smashed the whole system,” Deshpande noted. “But if you break the proton, at that instant you destroy the orbital motion of the gluons, so you can’t study it. Instead we want to probe the process more delicately without destroying the proton.” An electron from an electron-ion collider with high enough luminosity, or collision rates, would be just the kind of precise probe scientists need to tease out these subtle contributions to proton spin.
Gluon saturation
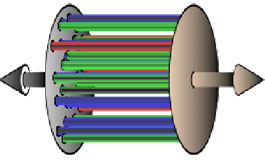
A schematic illustration of the stage just after two heavy ions collide, with relativistic effects making the receding ions appear as flattened disks. When the ions collide, they pass through each other and the gluons interact to form "flux tubes" (indicated as colored cylinders). This is the initial state of quark-gluon plasma (QGP), called a glasma, because it has properties that lie between those of the saturated gluon state of the ions before they collide — also known as a color glass condensate* — and the QGP.
A high-luminosity electron beam would also help physicists probe another interesting phenomenon they think occurs in particles moving ever closer to the speed of light. According to the theory that describes the interactions of quarks and gluons, known as quantum chromodynamics (QCD), as the firefly-like gluons “glow” longer, they should start to overlap, interact, and merge with one another, while new ones continue to pop out of the vacuum. Eventually, a balance is reached between gluons emerging from the vacuum and those recombining. The physicists call this steady state of maximal gluon concentration gluon saturation, or color glass condensate.*
Such a state of gluon saturation may have already been reached at RHIC — in the heavy ions before they collide.
“Those particles, gold ions, are moving at nearly the speed of light, and at light speed it is mostly gluons,” Venugopalan explained. “So really, what you have is two ‘walls’ of gluons colliding.” The quarks, which carry about half of the ions’ momentum, essentially move through one another, he said, while the dense fields of gluons are what stop and interact to begin the formation of the quark-gluon plasma.
The properties of those interacting gluon fields are therefore very likely linked to what’s observed in RHIC’s detectors.
Fingerprints in time
*Color Glass Condensate
Color is a type of charge that quarks and gluons carry as a result of their strong force interactions — which accounts for the term “chromo” in quantum chromodynamics (QCD). The word glass is borrowed from the term for silica and other materials that are disordered and act like solids on short time scales but liquids on long time scales. In the “gluon walls,” the gluons themselves are disordered and do not change their positions rapidly because of time dilation. Condensate means that the gluons have a very high density.
Scientists have learned a lot about the dense gluon fields by tracing RHIC’s particle paths back.
“If you look closely at the particles coming out of a gold-gold collision at RHIC, you find these correlations — a particle at one end of the collision seems to ‘know’ about another at the opposite end, and there’s a collective kind of flow to the particles,” Venugopalan said. “If you trace back the paths of these correlated particles, it’s telling you stuff that happened very early in the collision — how the flow was formed, and the details of the flow.”
In some ways, he suggested, it’s analogous to the way cosmologists learn about the very early universe by looking at the cosmic microwave background radiation. Those measurements of tiny fluctuations in the universe’s temperature 300,000 years after the Big Bang contain clues about what the universe looked like the instant after its birth, because certain aspects of its structure were “frozen out” at that instant leaving an imprint on the future measurements — and even on the large-scale structure we observe in the universe today, nearly 14 billion years after the Big Bang.
“Likewise at RHIC, we are looking at the spatial structure of the collision in ways that are very sensitive to the earliest moments of the collisions, when the gluons first interact and tiny fluctuations are in a similar manner frozen out and left as fingerprints,” Venugopalan said.
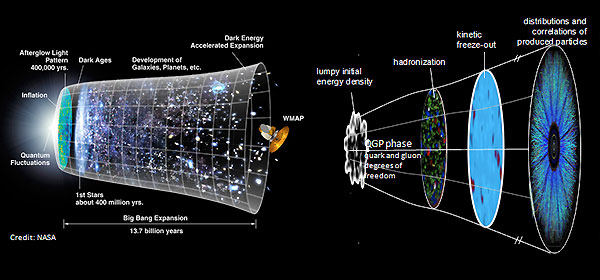
The particle tracks observed in RHIC's detectors (extreme right) contain fingerprints or clues that reflect the conditions very early in heavy ion collisions, when gluons in the colliding ions were just starting to interact. This is somewhat analogous to the way the structure we see in the universe today is a reflection of structural aspects "frozen out" very early in the history of the universe (left). In each frame, time runs from left to right, but over a span of 13.7 billion years in the left frame and only billionths of a second in the right frame. Scientists can learn a lot about these early conditions by looking back, but they'd also like to probe the earliest stage of ion collisions directly. An electron-ion collider would make that possible.
But with so much still unknown about the gluon fields — and so many of the unexpected characteristics of RHIC’s quark-gluon plasma dependent on them — scientists are eager to take a closer look.
The super bright electron beam of an electron-ion collider would allow them to do just that — send electron probes into light-speed ions to study the saturated gluon state directly.
“Before we built RHIC, we just wondered about the QGP — whether we could create it in a laboratory,” Deshpande said. “Now that we have created a QGP and we find it so much more interesting than we expected, we want to explore it more, to understand how its properties emerge. To get a complete picture, we need to explore in both directions — by looking back with heavy ion collisions, and by probing the structure and properties of the saturated gluon fields directly with an electron-ion collider.”
Collective behavior
The experiments would help reveal not just the femtoscale structure of individual particles, but also conglomerate structures and interactions among the constituent particles and fields.
“We are trying to go from a phase where we are studying the fundamental elements of the system — the particles — to understanding their collective behavior,” Venugopalan said. “It’s analogous to how this process led to the discovery of collective behavior like superconductivity in condensed matter physics. We are now trying to do condensed matter physics with quantum chromodynamics (QCD) — the theory that describes the strong force — the way physicists have been doing for many years with quantum electrodynamics — the theory of electromagnetism.”
If physicists can figure out how the gluon fields interact, how the quark and gluon spins and orbital motions coordinate with one another, how the hot quark soup thermalizes — reaches an equilibrium temperature of more than 4 trillion degrees Celsius — in a few trillionths of a trillionth of a second, who knows where it will lead?
eRHIC: Colliding Electrons with
Ions at RHIC
There are compelling reasons to build an electron-ion collider (EIC) at Brookhaven National Laboratory, not the least of which are the Lab’s rich physics history, accelerator expertise, and the existence of a fully functional and productive proton/heavy-ion accelerator/collider — that is the Relativistic Heavy Ion Collider (RHIC) — and its international collaborations of physicists eager to continue their explorations of matter. Adding an electron ring and other accelerator components to the existing accelerator complex would be a cost-effective, practical strategy for achieving the scientific goals of an electron-ion collider. More...
“This kind of curiosity and fundamental questions about electrons and protons and their interactions when present in large numbers are what drove us beyond the known electromagnetic interactions, and laid the foundation of condensed matter physics that is crucial for all the electronic gadgets we now use every day,” Deshpande said. “With an electron-ion collider, we could go from QCD as we know it today to a theory of condensed strongly interacting matter — and see where that takes us.
“But to do this, we have to go beyond the capabilities that the HERA collider had. It collided electrons with protons, but it didn’t have the heavy-ion beams, its proton beams were not polarized, and it did not have the brightness (luminosity) needed to explore all these pressing science questions that have only recently come to the fore.”
One thing is certain: If an electron-ion collider becomes a reality, what the physicists learn will offer deeper insight into what holds 99 percent of the matter in the visible universe together. That’s the percentage of everything we see around us — from stars to planets to our own physical forms — that gets its mass from protons and neutrons, and thus ultimately from the quarks and gluons governed by the strong force.
“At the most fundamental level,” Venugopalan concluded, “we are driven by our curiosity to learn more about what we are made up of.”
2012-2870 | INT/EXT | Newsroom