CFN Scientist Spotlight: Ashley Head Brings Surface Studies out of the Realm of Physics into Chemistry
interview with a CFN scientist
April 24, 2018
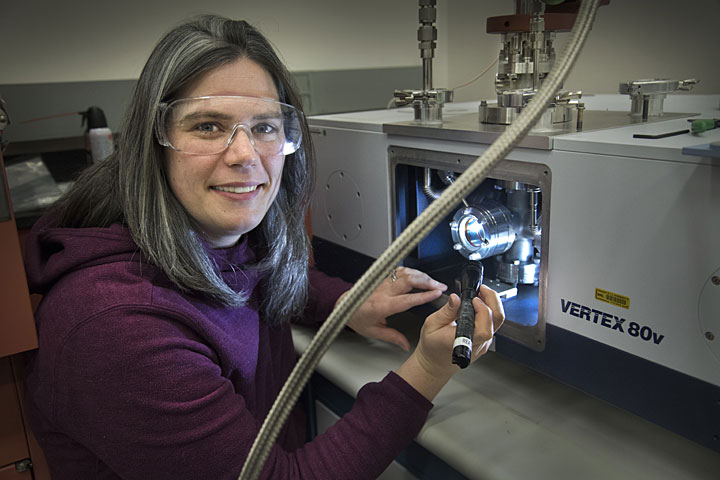
Chemist Ashley Head checks the alignment of a powder catalyst mounted inside the vacuum chamber of an infrared spectrometer at the Center for Functional Nanomaterials.
In the world of chemistry, surfaces and interfaces are exciting places. At such boundaries, atoms that make up one material interact with atoms of another material. Because of these interactions, chemical bonds are broken and new ones are formed. The chemical reactions result in the rearrangement of atoms, changing the fundamental properties of the materials involved. Ashley Head, a chemist in the Interface Science and Catalysis Group at the Center for Functional Nanomaterials (CFN)—a U.S. Department of Energy (DOE) Office of Science User Facility at Brookhaven National Laboratory—studies the interesting chemical processes and phenomena that take place on surfaces. An understanding of surface chemistry is relevant to many applications, such as designing efficient catalysts for fuel cells and developing more sophisticated gas masks for soldiers.
The mission of the Interface Science and Catalysis Group at the CFN—to discover and quantify atomic-scale processes and phenomena at interfaces, in real time and under operating conditions—very much aligns with your research. How did you come to join this group at the CFN?
I previously worked at DOE’s Lawrence Berkeley National Laboratory as a postdoctoral researcher, so I knew about the other DOE labs. In my research to understand what is happening on surfaces, one of the main experimental techniques I use is ambient-pressure x-ray photoelectron spectroscopy (AP-XPS). I knew that the CFN operates an AP-XPS beamline at Brookhaven’s National Synchrotron Light Source II (NSLS-II) [another DOE Office of Science User Facility], and I also knew the CFN was buying a lab-based AP-XPS system.
The CFN sounded like a fun place to continue doing my research, and I have really enjoyed my time here since starting in January 2018. Everyone has been very welcoming, and I am now in the process of getting some infrared spectroscopy equipment going and will start experiments soon. I am beginning to help users with infrared reflection absorption spectroscopy (IRRAS), another surface-sensitive technique that I use in my research. One user from Stony Brook University I am working with is pursuing electrochemistry research. Another user from Berkley Lab is studying the adsorption of carbon monoxide on gold surfaces.
How does ambient-pressure x-ray photoelectron spectroscopy work, and what do the resulting spectra tell you?
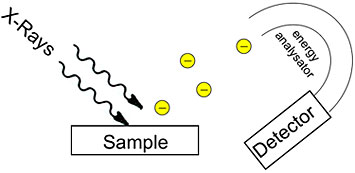
An illustration of how x-ray photoelectron spectroscopy works. Credit: Technical University of Munich
This technique takes advantage of the photoelectric effect, which was discovered by Albert Einstein. When you shine x-rays with a certain amount of energy onto a solid surface, electrons fly out. By measuring how fast they exit the sample, you can calculate the ionization energy, which gives you information about what element the electrons came from and what the atom is bound to (chemical functional group). For instance, you can determine that the electron came from a carbon atom that is bound to a nitrogen atom. AP-XPS not only tells you what elements are present but also their concentration and the electronic structure of atoms and molecules (how the electrons are configured in their shells).
Typically, XPS has to be done in vacuum because the electrons fly out from the sample. You cannot detect the electrons in an atmosphere of gas because they would run into gas molecules. But in the 1990s, Berkeley Lab scientists revived a technique from the 1970s to enable experiments in ambient-pressure conditions. On one side of the instrument, there is a chamber containing gas; on the other side, there is a chamber with lots of vacuum pumps. A system of lenses captures and focuses the emitted electrons to minimize their scattering through the gas phase. There is a really tiny entrance into the electron detector, which measure how fast the electrons are traveling. At first, AP-XPS was done at synchrotrons, including the Advanced Light Source [a DOE Office of Science User Facility at Berkeley Lab], because they have very intense x-rays and thus provide more electrons. It has since been developed for lab-based x-ray sources.
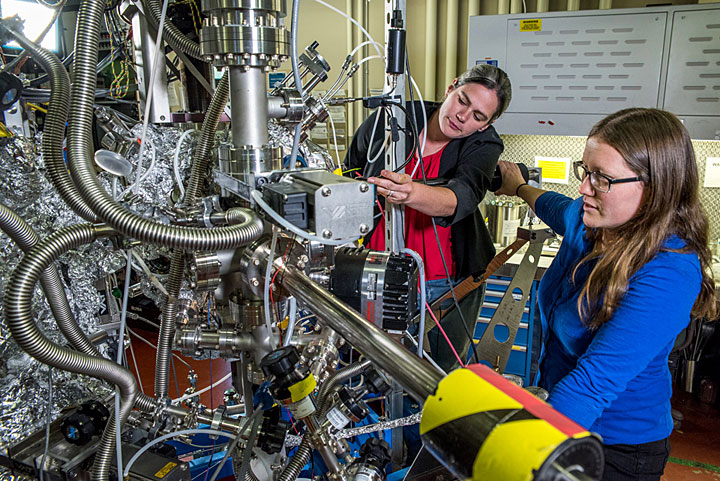
Ashley Head (left) with Berkeley Lab colleague Lena Trotochaud at the Advanced Light Source's AP-XPS beamline. Credit: Marilyn Chung/Berkeley Lab
You mentioned that infrared reflection absorption spectroscopy is another method you use to study surface chemistry. What information does it provide that ambient-pressure x-ray photoelectron spectroscopy does not?
IRRAS is a special kind of infrared spectroscopy in which you shine infrared light on a sample and then measure the amount of light that the sample absorbed. That measurement tells you what chemical functional groups are on a surface—the top couple layers of atoms. The reason this technique is so special is that it can be done at pressures of one atmosphere or higher. Compared to AP-XPS, IRRAS is more sensitive to the atoms on the surface. For example, AP-XPS may detect an alcohol group on a surface but cannot conclusively tell whether one, two, or three carbons are present. IRRAS can resolve such ambiguities raised by AP-XPS and provide additional complementary information.
I was actually the first to combine AP-XPS and IRRAS, as reported in a 2017 paper published in Surface Science. This combination had been a goal among scientists, especially because there has been a push in the surface science community to study systems that are technologically relevant to specific applications. In such systems, the chemistry gets more complicated, and spectral signals can overlap. Combining IRRAS with AP-XPS helps separate out those overlapping signals.
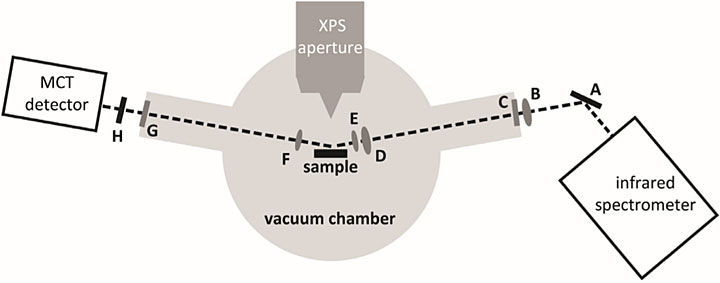
A schematic of the infrared (IR) beam path through the ambient-pressure x-ray photoelectron spectroscopy analysis chamber. A series of mirrors, lenses, windows, and polarizers (A–H) help focus the IR light onto the sample and the detector, which is made of the elements mercury, cadmium, and telluride (MCT). Source: Surface Science 665 (2017): 51–55.
The two techniques lend themselves to combination. They are compatible under similar operating conditions, and the instrumentation allows them to be integrated in a physical sense. The AP-XPS instrument I was using already had some windows in the right place where the IR light could come in. To start, I studied a very simple system—carbon monoxide on palladium surfaces. At the CFN, I will use this experience to help others incorporate IR spectroscopy in existing equipment, including low-energy electron microscopes and AP-XPS instruments.
How have you applied these surface-science techniques to your research?
One example is my work at Berkeley Lab. I was collaborating with a team from Johns Hopkins University, the U.S. Naval Research Laboratory, and the University of Maryland to investigate the chemistry of nerve agent simulants on the active components in gas mask filters. The filters that soldiers use are mostly carbon, like a water filter, but inside are metal-oxide particles.
While a postdoctoral researcher at Berkeley Lab, Ashley Head was part of a team using x-rays at the Advanced Light Source to study how gas filters handle nerve agent simulants. In this video, Head and fellow postdoctoral researcher Lena Trotochaud discuss how they use x-ray photoelectron spectroscopy to better understand the chemistry of metal oxides—key components in the filters—interacting with these simulants at the molecular level. Credit: Marilyn Chung/Berkeley Lab
Our study addressed several questions to better understand the molecular interactions that occur as various gases are adsorbed by the filters: Do these particles bind the nerve agent simulants or decompose them? If they decompose them, what do they decompose them to? What percentage of the simulant decomposes? Does the chemistry change based on the environment in which soldiers are wearing the gas masks? In other words, do conditions such as air pollution, diesel fuel exhaust, or water vapor alter the performance of the filters?
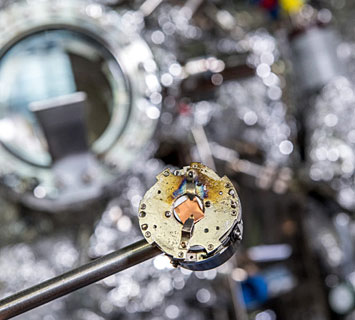
Copper is one of the materials used in gas mask filters to protect soldiers from toxic chemicals. Understanding the molecular interactions at the oxidized surface of this metal could help scientists design more advanced gas masks. Credit: Marilyn Chung/Berkeley Lab
We used AP-XPS to tackle these questions. One of the interesting things we learned is that copper oxide reacts at an unprecedented level—absorbing much of the nerve agent simulant and decomposing it to a much greater extent than some of the other metal oxides that have been studied. And the decomposition occurred through an uncommon mechanism. Our finding suggests it may be worth looking into ramping up the concentration of copper in the gas mask filters.
This fundamental research led to the U.S. Army Edgewood Chemical Biological Center purchasing a lab-based AP-XPS instrument to do these studies on real chemical warfare agents such as sarin.
What research will you be pursuing at the CFN?
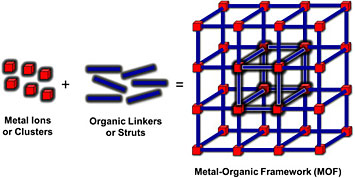
Illustration of metal-organic framework. Source: Coordination Chemistry Reviews 346 (2017): 101–111.
The Interface Science and Catalysis Group does a lot of research into nanoporous materials, and I plan to expand on that work through studying catalysis with metal-organic frameworks (MOF). These porous materials consist of metal centers linked together by organic ligands. They are used for many applications, including sensing, gas capture, energy storage, catalysis, and light harvesting. Scientists are very interested in MOFs because they are highly customizable, like LEGOS. Collaborators from UC Davis will be sending me samples of a zirconium-based MOF (UiO-66) to look at gas absorption mechanisms to gain insight into where gas molecules stick in the framework during catalysis.
The CFN recently purchased a lab-based AP-XPS instrument with a helium lamp that will enable me to do photoelectron spectroscopy using ultraviolet light. The spectra will reveal information about valence electronic structure of the MOFs under higher pressures. Because valence electrons are the highest-energy electrons in an atom, they are the most reactive.
In addition to studying MOFs, I will continue my research in atomic layer deposition (ALD)—a method to grow one layer of material at a time by flowing molecules over a surface. Intel uses this method to make computer chips, depositing individual molecular layers on the surface of silicon wafers. The idea with ALD is that you can grow films over any three-dimensional surface you want, to the exact thickness you want. In reality, the process is not quite that simple because defects form and unexpected side reactions occur. Understanding the deposition process is critical to producing the very pure (defect-free) thin films needed for fabrication of electronic devices and components.
In the past, I have used near AP-XPS to study the fundamental reactions occurring in ALD processes of titanium dioxide on ruthenium oxide surfaces. Thin films with this combination are good candidates for electrocatalytic anodes and capacitors in dynamic random-access memory (DRAM) storage. The experiments revealed important information about how pressure, temperature, and other factors affect these processes. I have also investigated the ALD of one mineral form of titanium dioxide (anatase) on a different form (rutile). These particular films are of interest to scientists because they could help smooth the rough surfaces and remove the defects and impurities that are common with growing anatase or rutile crystals on substrates of the same respective material.
At the CFN, I will be looking at the ALD mechanisms of other materials used in electronics. To start, I will study tantalum nitride, an inorganic chemical compound that is an attractive barrier material to separate interconnecting metal from silicon.
Your past work has relied on the use of x-rays from synchrotrons. Do you plan to use Brookhaven’s NSLS-II for your research?
NSLS-II will come into play for more difficult experiments in which I need to change the photon energy, need more intense x-rays for better signals, or want x-ray absorption data to understand the local structure around atoms. CFN materials scientist Anibal Boscoboinik of my group is the main CFN contact for the AP-XPS beamline at NSLS-II, so I will be working closely with him.
What capability do you wish you had for your research?
Adding time resolution to AP-XPS would greatly enhance our understanding of surface chemistry. I think it is the next major area where improvements are going to happen. Scientists often do steady-state experiments, in which they are looking at surfaces after the chemical reactions have reached equilibrium with the gas phase. Depending on how long-lived the intermediate products are, you may be able to catch them. For example, in the gas mask project, the intermediates live from several minutes up to a half hour, so we are able to see peaks in the signal and watch them disappear. But in other cases, the entire surface has already been transformed, and you do not know what happened to the surface during the reactions. You only have the starting and ending materials, and theory is needed to fill in the gap.
I definitely think a millisecond time scale is possible, and maybe even faster if looking at processes through laser pumping and probing. At this scale, you can get information about the chemical reaction itself and the intermediates. I hope to do some example studies in this area.
Did you always know you wanted to be a chemist? What was it about chemistry that drew you into the field, and how did you come to study surface chemistry in particular?
I initially wanted to be an archeologist. But when I was in fourth grade, my older brother told me that there was nothing left to be discovered. I had always looked up to him so he really crushed my dreams by telling me that, and I did not know what I wanted to do. I had always liked science because the same brother had electronic kits and science toys lying around when I was a kid, and I used to play with them. He ended up becoming an electrical engineer, and, while I was an undergraduate, he told me that I should become a chemical engineer because there were not enough of them at his workplace. The school that I was attending did not offer engineering. I figured I would at least do the chemistry part. I had always liked maps, and the periodic table seemed to me like a map of elements. Learning about the chemistry of the elements and all of the possible combinations excited me.
As an undergraduate studying chemistry at James Madison University, I took organic chemistry, which was all about carbon. Other elements seemed more fun and colorful, and so I was drawn to organometallic chemistry and inorganic chemistry. I also liked math, which I minored in. I had sort of been steering myself toward physical chemistry with inorganic systems.
After graduating, I worked for about a year in a dairy factory in Virginia, where I am originally from, in a quality control lab. I knew I did not want to do this job long term—I was making the same measurements day in and day out. Then I went to graduate school at the University of Arizona. For my PhD in chemistry, I did a lot of gas phase work. When I graduated, there were not many job opportunities in that area. I taught chemistry at a community college in Maryland for a year before taking a postdoctoral research appointment at Lund University in Sweden. For two years, I studied the ALD mechanisms of metal oxides, making use of Lund’s MAX IV synchrotron. From there, I went on to do more postdoctoral work—mainly the gas mask project—at Berkeley Lab.
Now I have a vision of combining inorganic chemistry and surface science. Surface science seems to be more in the realm of physics, but I feel like it can be brought more into chemistry.
I am so glad my brother talked me out of the archeology career. I like what I do now a whole lot more!
How do you plan on becoming involved in the CFN and Brookhaven Lab community?
Educational outreach is an area where I would like to be involved. I really enjoy doing science with kids. While I was at Berkeley, I taught science lessons once a month to minority-serving schools in impoverished areas in Oakland. In January, I was elected to the Users’ Executive Committee for Berkeley’s Advanced Light Source, and one of my goals on this committee is to help younger users of the synchrotron.
I am especially passionate about bringing more women into science. As the mother of a two-year old, I want to show other women that it is possible to have both a science career and children.
What do you want people to take away from your research?
I want as many people as possible to understand why I spend long hours in the lab doing experiments—what are the results good for, and how can they be applied to everyday life? I always put a lot of thought into how to better communicate science so that people can see the importance, whether I am writing a scientific manuscript, teaching kids about science, or telling my family what I do.
What do you hope to accomplish over the next few years?
I recently received the Distinguished Alumni Award for the College of Science and Mathematics from my undergraduate alma mater, James Madison University, for developing AP-XPS beyond traditional surface science. I hope to further develop it by building an ambient-pressure hard x-ray photoemission spectroscopy (AP-HAXPES) system. This system will enable me to conduct the same XPS experiments but with higher-energy x-rays. At this higher energy, I will be able to look at the bulk of materials instead of just their surfaces. AP-HAXPES will be especially useful in studying the chemistry taking place inside porous materials and at liquid-solid interfaces. These studies could help scientists better understand the electrochemistry of battery electrodes, the corrosion behavior of metals and alloys, and the environmental processes of minerals and sediments.
Brookhaven National Laboratory is supported by the Office of Science of the U.S. Department of Energy. The Office of Science is the single largest supporter of basic research in the physical sciences in the United States, and is working to address some of the most pressing challenges of our time. For more information, please visit science.energy.gov.
Follow @BrookhavenLab on Twitter or find us on Facebook.
2018-12891 | INT/EXT | Newsroom