Putting a New Spin on 1T Phase Tantalum Disulfide
Scientists uncover a hidden electronic state in a widely studied material
March 22, 2024
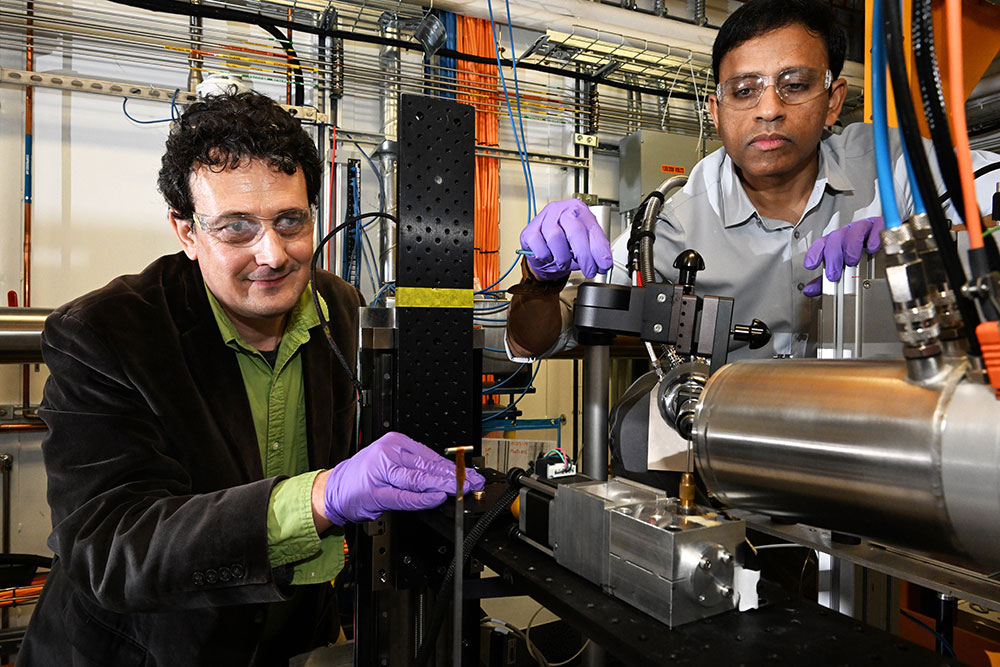
Emil Bozin (left) and Milinda Abeykoon (right) make experimental setup adjustments at the PDF beamline at NSLS-II (Kevin Coughlin/Brookhaven National Laboratory).
Research often unfolds as a multistage process. The solution to one question can spark several more, inspiring scientists to reach further and look at the larger problem from several different perspectives. Such projects can often be the catalyst for collaborations that leverage the expertise and capabilities of different teams and institutions as they grow.
For half a century, scientists have delved into the mysteries of 1T phase tantalum disulfide (1T-TaS2), an inorganic layered material with some intriguing quantum properties, like superconductivity and charge density waves (CDW). To unlock the complex structure and behavior of this material, researchers from the Jozef Stefan Institute in Slovenia and Université Paris-Saclay in France reached out to experts utilizing the Pair Distribution Function (PDF) beamline at the National Synchrotron Light Source II (NSLS-II), a U.S. Department of Energy (DOE) Office of Science User Facility located at DOE’s Brookhaven National Laboratory, to learn more about the material’s structure. While the team in Slovenia had been studying these kinds of materials for decades, they were lacking the specific structural characterization that PDF could provide.
The results of this collaboration, recently published in Nature Communications, revealed a hidden electronic state that could only be seen by a local structure probe like the pair distribution function technique. With a more complete understanding of 1T-TaS2’s electronic states, this material may one day play a role in data storage, quantum computing, and superconductivity.
A better vantage point provides a better view
When scientists study a material, they sometimes want to see the way atoms are arranged over the short range — a 10 nanometer scale — and sometimes they want to see how the patterns in an atomic structure repeat over the long range, such as a micrometer scale. The difference between these scales is comparable to looking at a few different buildings on one street versus the way buildings are arranged over several city blocks. Each of those tasks requires a very different vantage point. When studying the properties of a material, researchers may only be able to see certain behaviors at a specific length scale.
“We do several types of measurements at the beamline,” explained lead beamline scientist Milinda Abeykoon. “Usually, we use X-ray powder diffraction (XRD) to characterize the long-range order of a sample, but in this material, we suspected the coexistence of short-range ordered features that could lead to its interesting properties, so PDF was ideal for this kind of structural characterization. The beamline also has specialized equipment, like the combined cryostream and hot air blower setup, which was crucial for us to discover some of the subtle temperature-dependent features of this material over a very broad temperature range.”
“You can have a material that looks like an ideal, long-range ordered system when observed using XRD, but structural deviations at a shorter scale may be detected when PDF is used,” said Emil Bozin, a scientist leading the PDF research within the Condensed Matter Physics and Materials Science (CMPMS) Division at Brookhaven National Laboratory and one of the lead authors of this paper. “If we didn’t apply this technique, we wouldn’t have been able to see that there was actually a hidden short-range order in the system that all previously used probes had missed. There is an important local structural aspect to it.”
1T-TaS2: a layered material full of surprises
Transition metal dichalcogenides, or TMDs, are a class of materials constructed with atomically thin layers. TMDs feature a transition metal that sits between two layers of chalcogens, materials that contain oxygen, sulfur, and selenium. Each of these material layers is only one atom thick—one millionth the thickness of a strand of human hair. In the case of 1T-TaS2, a thin layer of tantalum is sandwiched between two sulfur layers. Each material has its own peculiar, layered structure, but when the layers are combined, electrons interact with each other in this different environment and create new properties.
TMDs have been studied for many decades as they display fascinating yet complex CDWs as they are cooled. CDWs are a particular long-range ordered alignment of charges that could be driven by different factors; in different TMD materials, layers stack in subtly different ways. How the structure orders itself creates a very specific system. 1T-TaS2 is special in many ways. Like other TMDs, it exhibits this CDW, but as opposed to the others that remain metallic, meaning they conduct electric current well, this particular system is actually insulating in its CDW state.
CDW is a quantum phenomenon that involves the movement of electrons that form a repeating pattern within a material. This arrangement affects the material’s electronic and structural properties, opening it up to different applications, including memory storage, sensor technology, and quantum computing.
Another notable feature of 1T-TaS2 is that it is a candidate material for quantum spin liquid. Quantum spin liquids are paramagnetic systems, meaning that the material has no long-range magnetic order. Due to quantum fluctuations, their spin never orders, even at low temperatures. These materials are characterized by quantum entanglement, which has brought them to the attention of researchers in the field of topological quantum computation.
“This is a concept that has been explored deeply in a theoretical sense,” said Bozin, “but there is little data on the actual system’s realization of these concepts. While we aren’t tackling this problem directly in our study, it’s one of the key features of this material that makes it so interesting. If it’s proven that this material’s theoretical spin liquid state can actually be stabilized, it opens up new possibilities in the world of quantum information science.”
Shining light on new phases
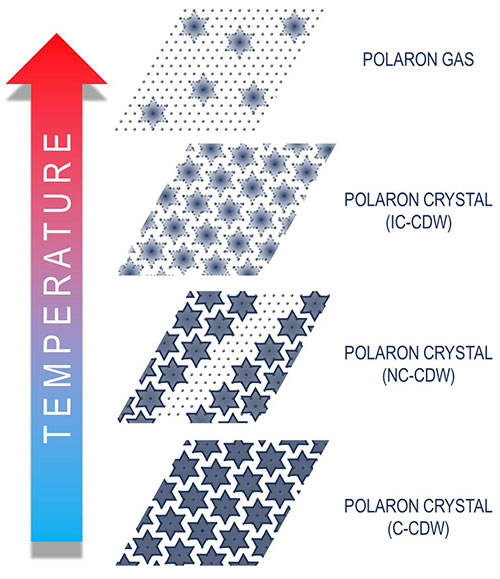
Evolution with temperature of polarons in tantalum layers of 1T-TaS2 through different CDW phases: commensurate (C), nearly commensurate (NC), and incommensurate (IC). When CDW is removed at high temperature, polarons are in a gas-like state.
“1T-TaS2 is not only interesting because of its potential in quantum computing. There are also applications in classical computing that are of more immediate practical interest,” said Dragan Mihailovic, head of the department for complex matter at the Jozef Stefan Institute in Slovenia and one of the lead authors of this paper. “We discovered that this material does something really extraordinary when exposed to very short pulses of light or electricity. These pulses can cause a change of the charge configuration within the CDW, which in turn leads to a large drop in electrical resistance. At low temperatures, these changes can enter a ‘metastable’ conducting state, which can be controllably switched back to the insulating state at will. This has practical applications in computing, like memory storage, which the team in Slovenia is already starting to explore with key players in the tech industry. The key advantages come from the fact that such devices exhibit sub-picosecond resistance switching times and have record-low dissipation in the atto-Joule range. Combined with excellent cycling and scaling properties, such ‘charge configuration memory’ devices based on 1T-TaS2 are very promising for all kinds of cryocomputing applications.”
“Using the PDF technique to explore the crystalline structure of 1T-TaS2 over a wide temperature range, we made several very surprising observations,” remarked Abeykoon. “The temperature of the material changes the electronic structure.”
As the temperature is lowered, the material enters the CDW state where the long-range order of the material begins to distort and change. Below 50 K — the temperatures where the application of quick light pulses results in a metastable state — the material exhibits an unexpected structural distortion that couples neighboring tantalum layers. This distortion may hold the key to achieving a long-lasting state created by pulses.
Conversely, warming the material above 550 K removes the CDW completely, which should result in an undistorted material.
“Surprisingly, short-range distortions similar to those seen at low temperature persist on a local scale at temperatures well above that of the CDW state,” explained Abeykoon. “This result provides an idea of what is driving the formation of CDW in this system.”
These high-temperature distortions originate from polarons, quasiparticles created by electrons as they move through the lattice structure of a material and interact with it locally. Above 600 K, the layered structure of the system begins to change irreversibly. It converts from a homogeneous stacking of one type of sulfur-tantalum-sulfur sandwich layer to a heterogeneous stack where every other sandwich layer changes its type. As the change happens, the number of polarons drops by 50%. This means that the polarons prefer only one type of sandwich layer — the one seen in pristine 1T-TaS2.
“This gives unmistakable proof for the existence of polarons well above the CDW ordering temperature, which has never been observed before,” said Mihailovic.
The charge ordering of this material — the pattern electrons create based on their density in different areas of a material — is driven by a completely different mechanism than one would traditionally expect. The ordering involves the crystallization of polarons into their own ordered state. This is similar to something known as a “Wigner Crystal,” which describes electrons arranged in a solid, crystalline state.
Understanding the complex electronic properties of this material and how to control them opens up a host of potential applications in electronics, sensing, and computing, but there is still so much more to learn. While these hidden states that are exhibited when hitting the material with ultra-fast laser pulses have been seen in the past, they have never been fully understood. The team is planning to decode the atomic structure and its relationship with ordered equilibrium structure. The temperature-dependent nature of the metastable state is still not fully understood. To fully realize the optical and electrical switching capabilities of this material for high-tech applications at warmer temperatures, researchers need to determine more details of this state.
“There are still several unexplored areas in this system,” said Bozin, “including the local structure. Our study has revealed that this system is actually much more complex, and it was already complex to begin with. There are secrets about this material that keep coming out, and they will continue to over the decades.”
Brookhaven National Laboratory is supported by the Office of Science of the U.S. Department of Energy. The Office of Science is the single largest supporter of basic research in the physical sciences in the United States and is working to address some of the most pressing challenges of our time. For more information, visit science.energy.gov.
Follow @BrookhavenLab on social media. Find us on Instagram, LinkedIn, X, and Facebook.
2024-21642 | INT/EXT | Newsroom