Precision Control of Beams in RHIC
December 5, 2011
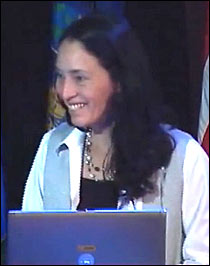
Michiko Minty leads the Instrumentation Systems Group in the Collider-Accelerator Department at BNL. Her focus has been on beam instrumentation and feedback-based beam control at RHIC. She is co-author of the book, Measurement and Control of Charged Particle Beams.
Abstract
The RHIC physics programs rely on particle beams of exquisite quality. For high luminosity this means maximizing the beam intensities and ensuring that the bunches of particles comprising the beams are as small and dense as possible. For experiments with polarized protons, the beam’s polarization must also be preserved. Within the past few years the quality of RHIC beams has been improved significantly: higher resolution measurements of the beam’s properties have allowed control of critical beam parameters using multiple feedback loops. Accelerator reproducibility guaranteed by feedback-based beam control has resulted in the highest proton beam polarization at highest beam energies achieved to date.
Introduction
Precision beam control in RHIC is challenging for a number of reasons; the sheer magnitude of the RHIC accelerators is such that there are thousands if not millions of potential error sources. These include static errors (such as relative lateral and/or rotational misalignments of the ~1750 superconducting magnets) and dynamic, or time-varying, errors with sources that include magnetic field variations due to persistent currents and hysteresis effects. A motivating example from RHIC run-9 without feedback showing the impact of diurnal variations is given below.
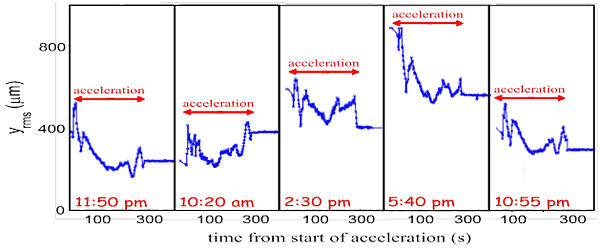
Vertical rms beam position for 5 different acceleration cycles (no feedback, run-9).
Plotted is the root-mean-square vertical beam position measured in one of the two RHIC accelerators as a function of time during the ~300s long proton energy “ramp” (time of acceleration) to 250 GeV. The five different plots correspond to different ramps executed at the indicated time of day illustrating the strong effect of outside temperature variations on the beam’s trajectories. Beam control based on direct measurements of and feedback on the beam’s parameters minimizes the impact of unknown error sources and guarantees constant and reproducible beam conditions.
Recent Developments
During RHIC run-11 each and every acceleration cycle was performed using simultaneous orbit, energy, tune, and coupling feedback [1]. Control of all these parameters relies on precise measurements of the beam’s transverse positions and betatron tunes (which is a measure of the frequency of oscillation of the beam, or particles within the beam, about the nominal beam trajectory and is a critical parameter with regards to beam stability). In addition to numerous modest hardware modifications [2] this was achieved primarily in two ways [3-4]. The first concerned providing better immunity to real position and tune variations caused by low frequency (~10 Hz) mechanical vibrations [6,7] of some of the main RHIC magnets. The second involved guaranteeing deterministic delivery and processing of data. Together these changes provided a >100-fold improvement in the resolution of the measured beam positions and >10-fold improvements of the beam tune and coupling parameters.
The first successful application of simultaneous tune and coupling feedback [8] was achieved during RHIC run-6 in a multi-institutional collaborative effort initiated by accelerator developments for the Large Hadron Collider. Robust and routine application of these feedback loops however became possible only after the above-mentioned improvements in the resolution of the input signals. In run-9 tune and coupling feedback were used during “ramp development” (e.g. first establishing accelerated beams to top beam energies), in run-10 for all ramps post-shutdown during commissioning prior to starting the physics program, and in run-11 continually for all acceleration ramps.
Meanwhile orbit feedback was developed to maintain reproducible beam trajectories as detailed analyses of the commissioning experiences from run-9 revealed that beam trajectory errors were the next leading cause for an acceleration cycle of insufficient quality to use as a reference for a subsequent ramp. Based entirely on existing infrastructure, this feedback loop uses measurements from ~320 beam position monitors together with ~30 million pre-computed model transfer functions to control ~230 corrector dipole magnets once per second during acceleration in each of RHIC’s two accelerators. The proof-of-principle for orbit feedback was demonstrated in run-10 [4,5]. In run-11 with continuous application during all acceleration cycles the algorithm was found to compete with a pre-existing so-called radial feedback, which matches the beam energy to the guiding magnetic fields by adjustments to the accelerating frequency. This interference was eliminated by constraining the average horizontal corrector dipoles strength to zero. In addition, the measure of the energy deviation, as derived from horizontal position measurements, is now more precise; it is now based on all position monitors located in areas sensitive to energy errors (e.g. in the collider arcs) as opposed to at just two locations used previously.
Shown below are data from RHIC run-11 acquired during normal physics operation with polarized protons accelerated to 250 GeV. Depicted are the rms vertical beam position (left, compare with Fig. 1) and the vertical betatron tune (right). Each plot contains a superposition of data from multiple acceleration cycles; on the far left of each plot, the initial parameter variations are visible followed immediately by the corrections made to these parameters by feedback just prior to beam acceleration. The reproducibility of the beam’s properties is demonstrated to be excellent.
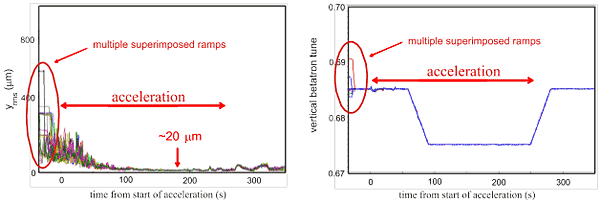
Superposition of vertical rms beam position (left) and betatron tune (right) data from multiple acceleration cycles (with feedback, run-11).
Impact on RHIC Operations
Feedback-based beam control in run-11 resulted in a significant increase in polarization transmission efficiency defined as the ratio of the polarization measured at top (250 GeV) and injection (~25 GeV) beam energies. Shown below are measurements from run-9 [9] of this ratio as a function of vertical betatron tune. The operating conditions from run-9 and run-11 are shown in red. With stability and reproducibility guaranteed by feedback, the vertical tune could be lowered during the critical part of the acceleration cycle much closer to the dangerous orbital resonance at a vertical tune of 2/3 and away from the spin depolarizing resonance at 7/10. With the figure of merit for statistical uncertainty in the physics program scaling as luminosity times the product of the square of the polarization of each beam (e.g. the fourth power of the polarization), the higher achieved polarization with feedback during acceleration was equivalent to about 14 additional weeks of RHIC operations (considering electrical operating costs only, or $3.5M) for the same level of statistical uncertainty in the physics program.
In a special dedicated and critical experiment executed to calibrate the RHIC polarimeters, the now nominal complement of feedback loops (orbit, energy, tune, and coupling) was applied together with chromaticity feedback [1]. The experiment involved polarization measurements made at 100 GeV before acceleration to 250 GeV and again after deceleration under nominally identical conditions back to 100 GeV. Comparison of the polarization measurements confirmed both the amount of polarization transmission loss and the calibration of the analyzing power used in the polarization measurements at 250 GeV [10]. The final feedback, chromaticity feedback, was used to maintain the desired energy dependence of the betatron tunes by adjustments to the accelerator sextupole magnets [11,12]. Offline analysis of the actions of the feedback loops (amount of corrections required to maintain the beam parameters) showed that this experiment would otherwise not have been possible in the allotted time without use of the complete suite of feedback loops.
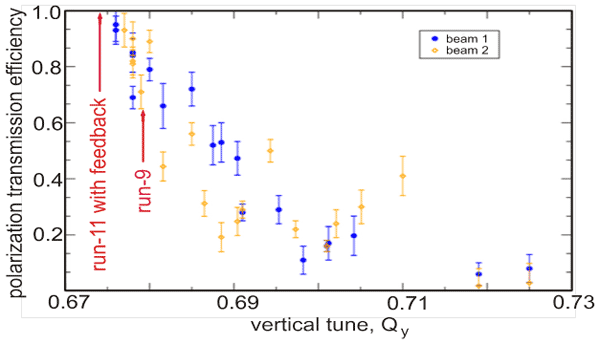
Ratio of polarization after to before acceleration to 250 GeV as a function of tune measured during RHIC run-9 [9] with operating conditions from run-9 and run-11 highlighted.
Summary
Improved measurement precision has contributed directly to improved control of the beam’s properties and to the successful application of routine orbit, energy, tune, and coupling feedback in RHIC. The reproducibility of critical beam parameters obtained with and without feedback-based beam control is given below. The level of stability now achieved is at the limits of the beam instrumentation. Overall, ramp development efficiency (the time required to establish beams to full energies with a new optic and/or change in particle species) has been reduced from several days (<2009), to one 8 hour shift (2009) down to 1 ramp or about 2 hours (2011); we estimate that accelerator availability has increased by ~ 1 week per fiscal year due to the improved reproducibility afforded by use of feedback-based beam control in RHIC. Precision control of the beam’s parameters has also expanded the parameter space accessible during acceleration resulting in a ~25% relative increase in each of the two proton beam’s polarization at 250 GeV. Simultaneous orbit, energy, tune, coupling, and chromaticity feedback were essential for realization of acceleration/deceleration ramps. We believe this achievement of feedback-based control of this complete set of the beam’s observables to be a world’s first in high energy colliders.

Level of stability achieved in run-9 without feedback and in run-11 with feedback during physics operation with 250 GeV polarized protons in RHIC.
References
- M. Minty et al, Precision beam instrumentation and feedback-based beam control at RHIC, Proc.2nd Intl. Part. Acc. Conf., San Sebastian, Spain (2011).
- M. Wilinski et al, Improvements for operational baseband tune and coupling measure-ments and feedback at RHIC, Proc. 2010 Beam Instr. Workshop, Santa Fe, NM (2010).
- R. Michnoff et al, RHIC BPM average orbit calculations, Proc. 2009 Part. Acc. Conf., Vancouver, Canada (2009).
- M. Minty et al, Global orbit feedback at RHIC, Proc.1st Intl. Part. Acc. Conf., Kyoto, Japan (2010).
- V. Ptitsyn et al, Slow orbit feedback at RHIC, Proc. 2010 Beam Instr. Workshop, Santa Fe, NM (2010).
- C. Montag et al, Observation of helium flow induced beam orbit oscillations at RHIC, Nucl. Instr. Meth. A 564, 26-31 (2006).
- M. Minty, Effect of triplet magnet vibrations on RHIC performance with high energy protons, Proc.1st Intl. Part. Acc. Conf., Kyoto, Japan (2010).
- P. Cameron et al, Simultaneous tune and coupling feedback in the Relativistic Heavy Ion Collider, and possible implications for the Large Hadron Collider commissioning, Phys. Rev. ST Accel. Beams 9, 122801 (2006).
- M. Bai et al, First polarized proton collisions at a beam energy of 250 GeV in RHIC, Proc. 2009 Part. Acc. Conf., Vancouver, Canada (2009).
- H. Huang et al, RHIC polarized proton operation and highlights, Proc.2nd Intl. Part. Acc. Conf., San Sebastian, Spain (2011).
- A. Marusic, M. Minty, and S. Tepikian, Chromaticity feedback at RHIC, Proc.1st Intl. Part. Acc. Conf., Kyoto, Japan (2010).
- M. Minty et al, Simultaneous orbit, tune, coupling and chromaticity feedback in RHIC, Proc. 2011 Part. Acc. Conf., New York, NY (2011).
- M. Minty et al, Precision beam instrumentation and feedback-based beam control at RHIC, Proc.2nd Intl. Part. Acc. Conf., San Sebastian, Spain (2011).
- M. Wilinski et al, Improvements for operational baseband tune and coupling measure-ments and feedback at RHIC, Proc. 2010 Beam Instr. Workshop, Santa Fe, NM (2010).
- R. Michnoff et al, RHIC BPM average orbit calculations, Proc. 2009 Part. Acc. Conf., Vancouver, Canada (2009).
- M. Minty et al, Global orbit feedback at RHIC, Proc.1st Intl. Part. Acc. Conf., Kyoto, Japan (2010).
- V. Ptitsyn et al, Slow orbit feedback at RHIC, Proc. 2010 Beam Instr. Workshop, Santa Fe, NM (2010).
- C. Montag et al, Observation of helium flow induced beam orbit oscillations at RHIC, Nucl. Instr. Meth. A 564, 26-31 (2006).
- M. Minty, Effect of triplet magnet vibrations on RHIC performance with high energy protons, Proc.1st Intl. Part. Acc. Conf., Kyoto, Japan (2010).
- P. Cameron et al, Simultaneous tune and coupling feedback in the Relativistic Heavy Ion Collider, and possible implications for the Large Hadron Collider commissioning, Phys. Rev. ST Accel. Beams 9, 122801 (2006).
- M. Bai et al, First polarized proton collisions at a beam energy of 250 GeV in RHIC, Proc. 2009 Part. Acc. Conf., Vancouver, Canada (2009).
- H. Huang et al, RHIC polarized proton operation and highlights, Proc.2nd Intl. Part. Acc. Conf., San Sebastian, Spain (2011).
- A. Marusic, M. Minty, and S. Tepikian, Chromaticity feedback at RHIC, Proc.1st Intl. Part. Acc. Conf., Kyoto, Japan (2010).
- M. Minty et al, Simultaneous orbit, tune, coupling and chromaticity feedback in RHIC, Proc. 2011 Part. Acc. Conf., New York, NY (2011).
2011-2757 | INT/EXT | Newsroom