- Home
-
Research Groups
Division Groups
- Artificial Photosynthesis
- Catalysis: Reactivity & Structure
- Electrochemical Energy Storage
- Electron- and Photo-Induced Processes for Molecular Energy Conversion
- Neutrino and Nuclear Chemistry
- Surface Electrochemistry and Electrocatalysis
Associated Groups
- Catalysis for Alternative Fuels Production
- Nanostructured Interfaces for Catalysis
- Structure and Dynamics of Applied Nanomaterials
- People
- Operations
- News
- Events

Neutrino and Nuclear Chemistry
Neutrino study currently is a very hot topic in science. Why? Because there are strong indications from several experiments that the neutrino has new properties, such as non-zero rest mass (so-called "New Physics") that are not included in standard theories of elementary particles. Theory may have to be recast to explain the experimental results.
Neutrinos from Nature
The neutrino was proposed by Wolfgang Pauli in 1930, but it took 26 more years before the neutrino was actually discovered (detected). Pauli proposed the existence of the neutrino as a solution to a frustrating problem in nuclear beta-decay that was studied in the laboratory, namely that examination of the reaction products always indicated that a variable amount of energy was missing. Pauli concluded that the products must include a third “almost invisible” particle, one which didn't interact strongly enough for it to be detected. Enrico Fermi called this particle the “neutrino” (for the "little neutral one"). In 1956, Reines and Cowan discovered the neutrino interactions in their experiments at a nuclear reactor (Reines was jointly awarded the Nobel Prize in physics in 1995).
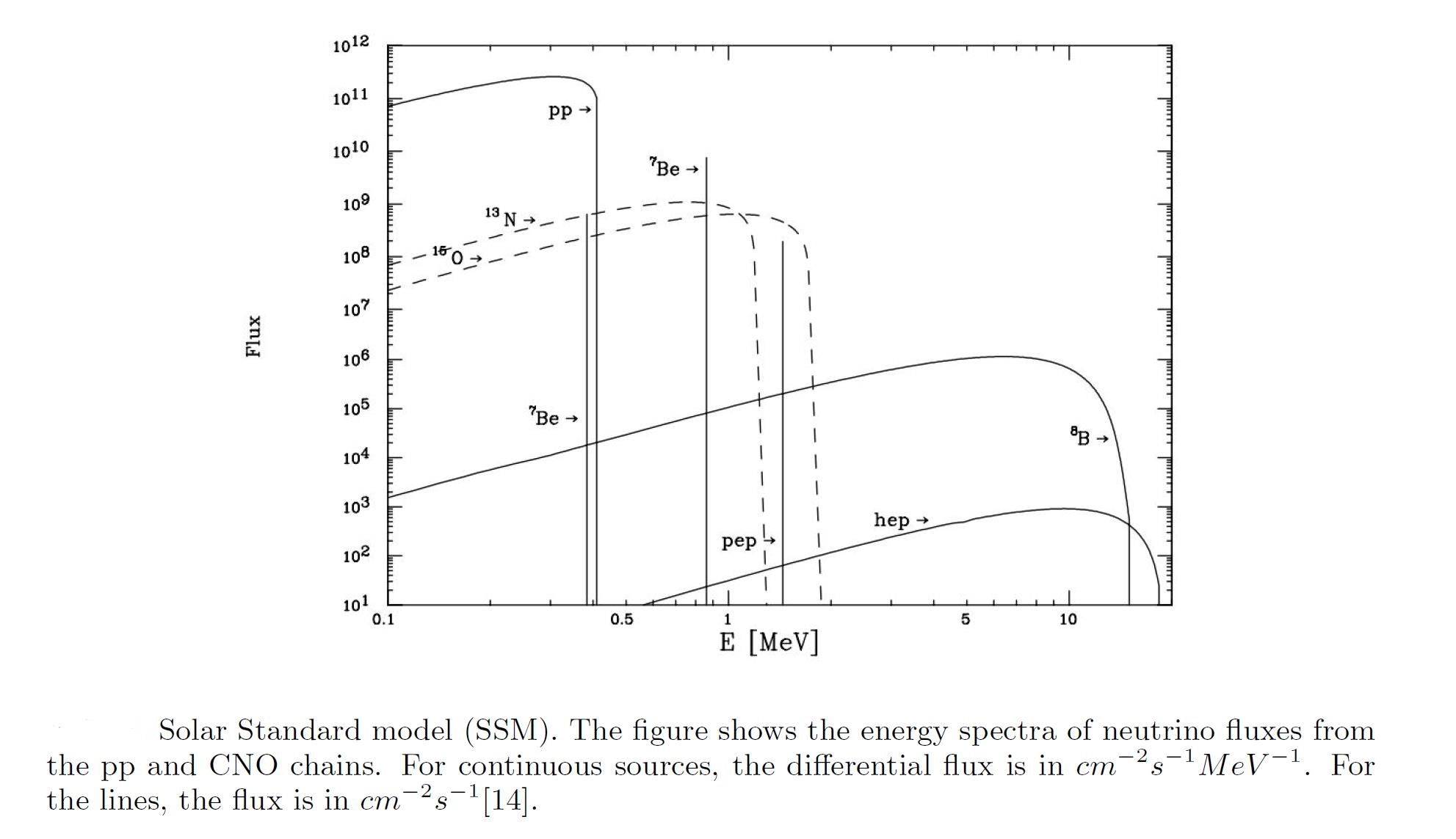
Today, neutrinos are known to be tiny mass, neutral elementary particles, which interact with matter via the weak nuclear force. The weakness of the weak force gives neutrinos the property that matter is almost transparent to them. It is fascinating that the neutrino, which was “invented” to solve a laboratory nuclear physics problem, must also be invoked to explain energy production in our sun and in all other stars. We now know that nuclear fusion and decay processes, which occur within the stellar core, produce copious amounts of neutrinos. As shown in the figure, the standard solar model predicts that these reactions produce several groups of neutrinos, with differing fluxes and energy spectra.
Neutrinos from Man-made Sources
Two most imperative artificial sources of manmade neutrinos are (1) fission reactors that utilize enriched uranium or plutonium isotopes to produce electron-type antineutrinos from beta decays of fission fragments, and (2) proton accelerators that can be operated at different modes to produce muon neutrinos (or antineutrinos) from pion decays in flight.
Reactor Neutrinos
These fission fragments generated from fission reactions of heavy nuclei (commonly U-235 or Pu-239) are unstable leading to a cascade of beta decays in the process of transforming into stable nuclei. The beta decay of the fragments is the source of the electron-type antineutrinos. The fission energy and yield depend on the composition of fuel and type of reactors. On average, each fission yields about 200 MeV of energy with ~6 electron antineutrinos [https://arxiv.org/pdf/1605.02047.pdf]. You can then calculate the number of neutrinos produced per second from the power output of the reactor.
The neutrino experiments use the number of neutrinos and their energy distributions to calculate the probability that a neutrino will interact in the detector as a function of distances from its originated source. Some challenges for the precision of the model calculation could be additional fissional isotopes (e.g. U-238, Pu-239, or Pu-239 generated from a U-235 nuclear fuel) or different fission fragments produced from each nuclear fission reaction, which need to be taken into account.
Accelerator Neutrinos
The most common neutrino beam is produced from proton accelerator (synchrotron). The energy of the protons, along with the geometry of the beamline, determines the energy of the neutrinos. Secondary pions (along with many other particles) are first produced from the target materials bombarded with highly energetic protons, then selected by their charges (positive for neutrinos and negative for antineutrinos) using magnetic fields (horns) for decays. These charged pions (0.026 μs) are then focused into a collimated beam for decaying into muons and (anti)neutrinos. The muons (2.2 μs) then further decay to electron and positron and more neutrinos.
Very pure beam of muon-neutrinos (or muon-antineutrinos) with predictable neutrino energy can be obtained from the beam parameters of proton accelerator and has been widely used for a variety of nuclear and particle physics experiments to study neutrino oscillations and neutrino flavors.
Methods of Neutrino Measurements at Neutrino and Nuclear Chemistry Group
Radiochemical Neutrino Detectors
The field of solar neutrino research had its birth in the BNL Chemistry Division, where Raymond Davis and colleagues developed a radiochemical method to separate and detect the few radioactive atoms formed by capture of solar neutrinos in a huge target. This first solar neutrino experiment, in the Homestake Mine in South Dakota, used the isotope, 37Cl, as the target in a 100,000-gallon tank full of perchloroethylene.
Neutrino capture on the 37Cl, with an energy threshold of 0.814 MeV, produces radioactive 37Ar, a gas, which is removed from the target, purified, and counted. The results of this experiment revealed a "solar neutrino problem": The number of measured solar neutrinos was only about one-third of the value predicted from solar theory.
Another radiochemical neutrino detector was developed at BNL, using 71Ga as the target. Neutrino capture on the 71Ga produces radioactive 71Ge with an energy threshold of 0.233 MeV. This 71Ge can be removed from the liquid target in the form of gaseous GeCl4, chemically purified, and converted to GeH4 gas for counting. Two gallium detectors based on this scheme were constructed and operated. The NCC Group participated in GALLEX at the underground Gran Sasso National Laboratory in Italy, where 30 tons of gallium in the form of a 100-ton aqueous solution of gallium trichloride served as the target.
Water Cerenkov Neutrino Detectors
When light travels through water (the refractive index of 1.33 at room temperature), its speed is slowed down by about 3/4 of that in vacuum. On the other hand, charged particles are not affected by the refractive index and could travel faster than light in water. These high-energy charged particles could then emit Cerenkov radiation (similar to an optical, sonic boom) along the path traveling through water.
Neutrinos interact with atoms in water to produce high-energy electrons or muons that can travel faster than the speed of light through water and produce Cerenkov radiation that can be detected by photosensors. The axis of the Cerenkov cone gives the direction of the particle, and the light yield of Cerenkov radiation gives the particle energy. Only charged particles with β > 1/n can be detected: this gives a threshold total energy of about 0.8 MeV for electrons, 160 MeV for muons and 1.4 GeV for protons and neutrons.
SNO uses heavy water as the detector target to detect different flavors of neutrinos. The deuteron in the D2O in SNO makes it unique among neutrino detectors, since it can observe all three neutrino flavors. The electron neutrino is the only flavor that can convert the D into 2 protons + an electron. This electron provides the signal for the so-called "charged current" (CC) neutrino reaction. In the meantime, all three neutrino flavors are also equally effective in breaking apart the D into its constituents, a proton + a neutron.
This neutron provides the signal for the "neutral current" (NC) interaction. If SNO was to measure the NC rate to be greater than the CC rate, this would be definitive proof, a "smoking gun", for the existence of neutrino oscillations. The cause of the solar neutrino problem would be the transformation of some of the solar electron neutrinos into the other flavors. The physics community is excited by this prospect for new physics. A spin-off of such a result is that massive neutrinos could account for the "missing mass" that is required for a closed universe.
Applications: SNO
Liquid Scintillator Neutrino detectors
Organic liquid scintillator detectors provide the necessary stopping power and sensitivity required for neutrino detection and have played a key role in the understanding of neutrino physics since Reines and Cowan. Metal-loaded Liquid Scintillators (M-doped LS) and unloaded liquid scintillators (LS) that are required for successful neutrino and antineutrino detections are being extensively developed at BNL. The work in the development of LS, especially with metal loading, has generated great interest in the fields of particle and nuclear physics, and other scientific communities.
Many interests of exploring the possibility of developing a variety of metal-loaded LS in proposed new experiments, not only for detection of sub-MeV neutrinos, super-nova neutrino and dark matter searches, but also, for application of national security and reactor monitoring have been summarized in [link to BUCK and YEH, Phys. G: Nucl. Part. Phys. 43 093001 (2016)]. There are only a very few groups in the world capable of conducting the type of development that applies (nuclear) chemistry to forefront physics experiments and scaling from in-house R&D to ton-scale production.
We have developed and refined recipes for preparing the M-doped LS, involving organic carboxylates and other complexing ligands to complex the metal in scintillating solvents, such as PC, DIN, PXE and LAB. These recipes are being translated into processes that can be applied at the multi-ton chemical scale. This research at BNL focuses on these chemical questions, including determination of the chemical species that constitute the M-doped LS. Key chemical and nuclear-chemical characteristics are (a) long-term chemical stability, (b) high optical transparency, (c) high light production by the scintillator, and (d) ultra-low impurity content, mainly of natural radioactive contaminants, such as U, Th, and Ra, and of chemical contaminants that can reduce the light output or light transmission or alter the chemical stability of organocomplex in scintillator.
We also are doing R&D on the determination and reduction of the levels of background impurities, and on the chemical compatibility of the organic LS with transparent plastics, such as acrylics, that will be the material used to construct the detector vessels. Some assay methods involve using low-level spikes of uranium, thorium, or radium and other radiotracers.
Current works focus on the preparations with varied concentrations (% in weight) of different metals, as target or detection materials, loading in the organic liquid scintillator for a variety of nuclear and particle physics experiments: (a) 200-ton, 0.1% gadolinium in LS (Gd-doped LS) to detect antineutrinos and measure the theta-13 mixing angle at Daya Bay, (b) 860-ton, 0.5% or more DBD targets (tellurium, previous neodymium) in LS (Te-doped LS) to search for neutrinoless double beta decay in 130Te at SNO+ (SNOLAB in Sudbury), the successor to SNO to use most of the physical facilities of the SNO experiment, (c) 125-ton, 8% indium in LS (In-doped LS) to measure the lowest energy solar neutrinos from the pp, pep, and 7Be solar branches in the Low Energy Neutrino Spectroscopy (LENS) either at the Kimballton Underground Research facility or other Deep Underground Laboratories, (d) 5-ton, 0.1% lithium in LS (6Li-doped LS) for precision measurement of neutrino oscillation and spectrum at short baseline (PROSPECT) at ORNL and (e) 20-ton, 0.1% ultrapure gadolinium in LS (Gdm-doped LS) as the veto detector for dark matter search (LZ) at SURF, SD.
Note that many tons of M-doped liquid scintillators for both PROSPECT and LZ experiments were produced and stored at BNL before the occupancy readiness of each experiment to receive scintillators for detector filling. This approach of using a centralized scintillator production center with a storage facility (capable of 100 tons or more) along with viable shipment schemes is cost- and schedule-effective that also minimizes onsite space- and labor-requirement, which are very challenge in confined space, in particular underground, for many large-scale scintillator experiments.
Water-based Liquid Scintillator Detector (WbLS)
A pure, many tens of kilotons of liquid scintillator has great sensitivity for sub-MeV neutrinos and dark matter searches and can push the current limit of proton decay lifetime (~1033 yrs) by an order of magnitude lower. However due to the cost, ES&H and chemical safety, this large pure LS detector is currently not a favored choice. The success of water-based liquid scintillator will provide a new generation, cost-effective and environmental benign, detection medium that could make the large PDK+ detection affordable and largely reduce chemical usage and waste for kilotons of neutrino detectors. This also has great potential in national security application and reactor monitoring.
The main motivations of developing the water-based liquid scintillator are (1) to optimize the ES&H and chemical safety on DOE missions by reduction of large quantity (tens of kT) organic liquid scintillator, (2) to create a significant cost-saving technology for future large-scale physics experiments, and (3) most importantly, to generate a novel detection medium that is capable of both scintillation and Cerenkov detection channels to explore low-energy physics below threshold.
New applicable detection medium to enhance national security and to replace the current scintillation cocktails further motivates the interest. BNL initiates the development of a mass-producible recipe for WbLS that (1) has been stable for years of storage at liter-scale since synthesis in laboratory environment, (2) is proficient in loading of varied target metals (particularly hydrophilic elements that is difficult to be loaded into non-polar organic liquid scintillator), and (3) capable of producing (tunable) scintillation light with fast decay time and emission region of interest.
Material Compatibility Program
The material leaching in and deterioration by liquid are the great concerns of neutrino experiments. BNL group has the expertise and facilities to carry out compatibility tests in different detection mediums. R&D procedures for (1) performing aging tests to speed up the test time in response to the data-taking lifetime of experiment; (2) measuring temperature-activation coefficient (Q10) to precisely predict the material behaviors and liquid impacts; and (3) building a material database per liquid to benefit the science community, are under development.
BNL is the leading institute of material testing for SNO, LENS, SNO+, Daya Bay, PROSPECT, LZ, AIT/NEO and the designated group for previous water-phase development for Long Baseline Neutrino Experiment (LBNE).
This program will provide a better understanding of material leaching behaviors in water and in liquid scintillator to ensure the detector achieving its goals at planned lifetime. Standard QA/QC procedures for material selections and cleaning are developed. A database with detailed vendor and model information is under construction that could benefit all science community in current and future experiments. A simulated model can also be built to incorporate any purification schemes for a clear assessment with purification facility, which could lead to significant cost saving.