Capturing the Chemistry of Light-Activated Cancer Drugs with Ruomei Gao
interview with a CFN user
May 21, 2021
Ruomei Gao is an associate professor in the Chemistry and Physics Department and member of the Institute for Cancer Research and Education (ICare) at the State University of New York (SUNY) College at Old Westbury, a teaching-intensive, minority-serving liberal arts college. Gao’s research focuses on light-induced chemical reactions (photochemistry) and the chemical composition of matter (analytical chemistry). In particular, she studies photosensitization of porphyrins and thiopurines to produce reactive oxygen species for cancer therapy and prevention, respectively. Since 2014, she has been using facilities at the Center for Functional Nanomaterials (CFN)—a U.S. Department of Energy (DOE) Office of Science User Facility at Brookhaven National Laboratory—to investigate two primary processes of photosensitization. She holds a doctorate in analytical chemistry from the University of Science and Technology of China, a master’s in analytical chemistry from Hebei University in China, and a bachelor’s in chemistry, also from Hebei University.
Which photosensitization processes does your lab study?
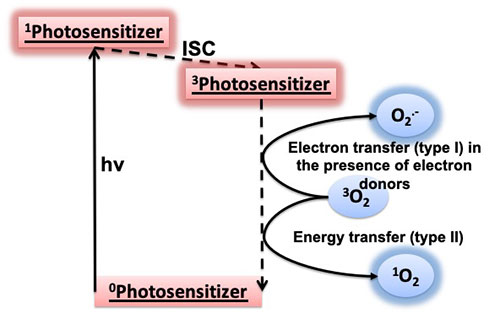
A schematic showing type I and II photosensitization processes. A photosensitizer at the ground state (0Photosensitizer) can be excited to its singlet state (1Photosensitizer) upon light irradiation (hν). This singlet state converts to a lower-energy triplet state (3Photosensitizer) via a nonradiative process called intersystem crossing (ISC). In the presence of oxygen in its stable triplet form (3O2), which is the oxygen found in air, the excited triplet photosensitizer can transfer electrons to oxygen to produce superoxide radicals (O2−) or transfer energy to oxygen to produce singlet oxygen (1O2).
In my lab, I like to say we play with three things: light, oxygen, and photosensitizers. A photosensitizer is a light-absorbing compound. Usually, it’s an organic (carbon-containing) compound with color but sometimes without. When you shine light on the photosensitizer, it becomes excited. In the presence of oxygen, two basic processes can occur. In one process, which we call type I, the excited photosensitizer transfers electrons to oxygen to produce a reactive oxygen species called superoxide radicals. This process usually requires the presence of some electron-donating compounds. In another process, which we call type II, the excited photosensitizer transfers energy to oxygen to produce singlet oxygen, another reaction oxygen species.
How do these processes and the resulting products relate to cancer therapy and prevention?
Superoxide radicals can be good or bad. They play a role in biological metabolism but may be bad because they can cause biological damage, especially if they convert into other reactive species. For example, if a superoxide is in “Fenton-like conditions” (in the presence of hydrogen peroxide and iron) it will convert into extremely reactive hydroxyl radicals. Hydroxyl radicals are the most active type of radical, meaning they can interact with or kill anything.
That’s what we think may be happening with thiopurine prodrugs including azathioprine, 6-mercaptopurine, and 6-thioguanine (6-TG). A prodrug is an inactive precursor compound that, after administration to a patient, gets converted in the body into a pharmacologically active drug. These three thiopurine prodrugs—which are used to treat cancer as well as autoimmune disorders and inflammatory bowel disease—are metabolized to form 6-TG nucleotides. 6-TG is an analog to guanine, one of the four chemical bases found in DNA. In 6-TG, the oxygen atom is replaced with a sulfur atom.
Patients taking these thiopurine prodrugs over several years have an increased risk of developing cancer because the final metabolite (6-TG nucleotides) gets incorporated into their DNA.
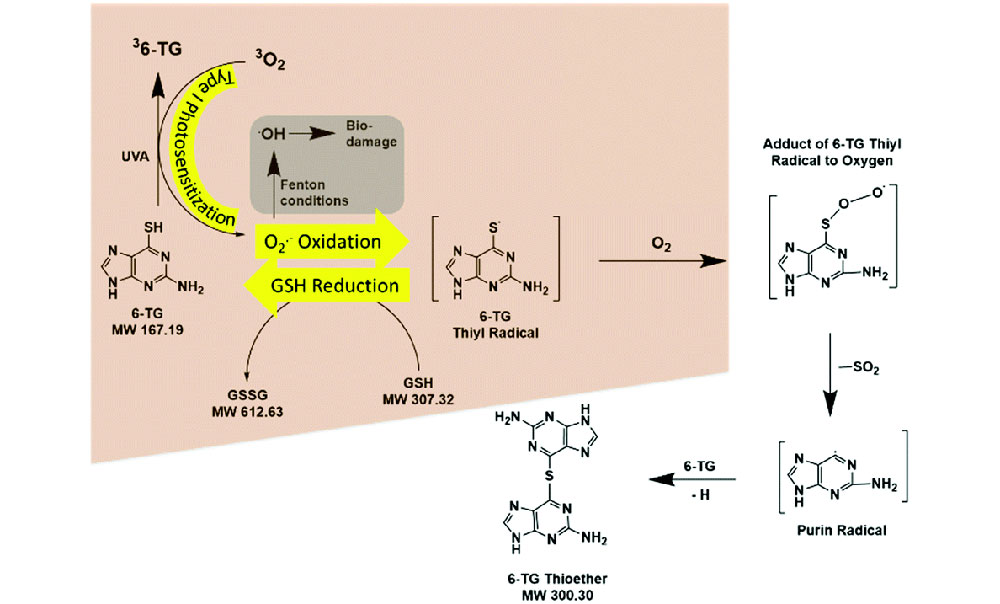
A schematic showing the type I photosensitization of the drug 6-TG induced by ultraviolet A (UVA) light in the presence (highlighted in peach) and absence of gluthathione (GSH). The gray box shows how superoxide radicals convert into extremely reactive hydroxyl radicals (•OH) under "Fenton-like conditions." Hydroxyl radicals have an even higher risk of causing biological damage than that induced by the well-studied type II reaction. Research published in Physical Chemistry Chemical Physics 9 (2021).
Scientists think this side effect is related to the reactive oxygen species generated when the drugs absorb ultraviolet A (UVA) light. Most of the studies to date, including ours, have focused on the type II process (production of singlet oxygen). But we recently explored the type I process (production of superoxide radicals) with 6-TG and found the production of superoxide radicals increases 10-fold in the presence of gluthathione (GSH)—a very common compound in biological systems. 6-TG is ready to be oxidized by reactive oxygen species, while GSH can restore oxidized 6-TG to its original, active state. 6-TG acts as a continuing source of oxidants via type I photosensitization-superoxide oxidation-GSH reduction cycles.
Singlet oxygen is known to kill cancer cells. The energy-transfer process that produces singlet oxygen is what photodynamic therapy is based on. In photodynamic therapy, patients receive drugs that only work after they’ve been activated by certain wavelengths of light (photosensitizers), usually from a laser. A challenge in photodynamic therapy is that the singlet oxygen not only kills cancer cells but also healthy cells.
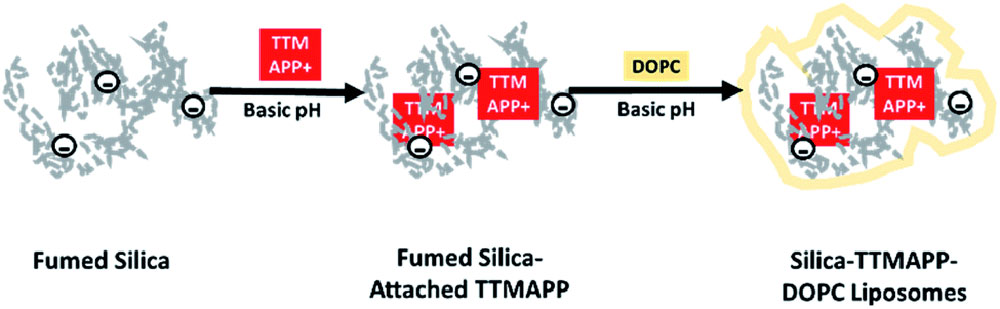
An illustration showing the process for creating the silica-porphyrin (TTMAPP) liposomes for targeted drug delivery via photodynamic therapy.
In our work, we’ve been using porphyrins as the photosensitizer to produce singlet oxygen. Porphyrins are colored organic compounds with a ring structure, and they absorb visible or near-infrared light. To try to improve the selectivity of photodynamic therapy, we leveraged one of the differences between cancerous and healthy cells: their pH. Cancer cells have an acidic (lower) pH than healthy cells, which have a slightly basic pH. We developed a photosensitizer that only works at lower pH. The mechanism is very simple. We attach the photosensitizer—positively charged porphyrin—to silica, or silicon dioxide (SiO2), nanoparticles. We use silica because its charge changes with pH. At higher pH, silica is negatively charged. When silica and porphyrin attach, the porphyrin gets deactivated for photosensitization. We encapsulate the porphyrin-silica in a phospholipid-based drug carrier called a nanocomposite liposome.
As we demonstrated with prostate cancer cells, when the liposome encapsulant is placed in a lower pH, the silica nanoparticles become positively charged. The two positive charges repel each other, causing the liposome to collapse and the nanoparticles and porphyrin to separate. The released porphyrin is now free to produce singlet oxygen. We found that singlet oxygen production is five times higher in lower pH environments.
What prompted you to submit a user proposal to the CFN?
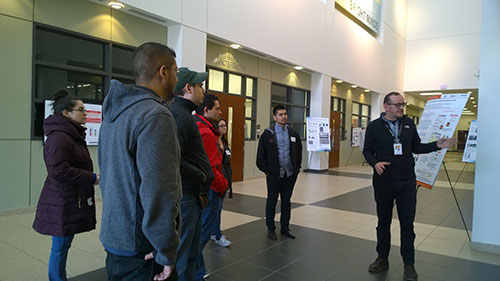
CFN materials scientist Mircea Cotlet—Gao's current host—gave a presentation to some of Gao's students during a tour of the CFN in 2017.
I learned about the CFN the first year I joined Old Westbury from a colleague who had collaborated with them for many years. Old Westbury is not a research institution, so our experimental facilities are limited. I told colleagues what capabilities I needed for my research and they connected me with Eric Stach [former leader of the CFN Electron Microscopy Group]; Kim Kisslinger and Fernando Camino, both staff members in the CFN Electron Microscopy Group; and Mircea Cotlet, Mingxing Li, and Fang Lu of the CFN Soft and Bio Nanomaterials Group. Without the capabilities and staff expertise at the CFN, I don’t think my publications on the photosensitization research would have been possible.
Which capabilities at the CFN have you been leveraging for your research?
It’s not only me who comes to the CFN; I also bring my students. We have been using a transmission electron microscope in the Electron Microscopy Facility and a dynamic light scattering instrument in the Advanced Optical Spectroscopy and Microscopy Facility to measure particle sizes. We want to know how the particle size changes when porphyrin is attached to silica. If the particle size is too big, it will be hard to distribute or deliver the particles as nanodrugs.
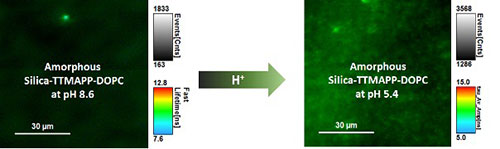
An illustration showing representative fluorescence images monitored at 560 nanometers (nm) upon irradiation (410 nm) of porphyrin in the dry layers of liposomes prepared at pH 8.6 (left) and pH 5.4 (right). The images were captured with the scanning confocal fluorescence lifetime imaging microscope at the CFN. The brighter green at pH 5.4 (compared to the darker green at pH 8.6) indicates more free porphyrin molecules have been released for singlet oxygen production, thus leading to more cancer cell death. Research published in RSC Advances, 10, 17094 (2020).
We have also been using a laser in the Advanced Optical Spectroscopy and Microscopy Facility to excite the photosensitizer and a near-infrared detector to measure the amount of singlet oxygen produced. Singlet oxygen generates an emission signal at 1270 nanometers, a wavelength of light in the near-infrared region. The quantity of singlet oxygen is related to how much photosensitizer you have and the intensity of the laser light. Normally, we put the photosensitizer in a cell—usually breast cancer cells—and vary these conditions to see their effect on cell death. I especially like the scanning confocal fluorescence lifetime imaging microscope, which is equipped with a camera that allows us to image the photosensitizer. In our lab at Old Westbury, we can measure absorption and fluorescence, but we don’t have the imaging capability.
What do you plan to work on next?
Right now, I have an active user proposal with the CFN, mainly to conduct laser experiments in Mircea’s lab to look at both processes—type I and type II—in a water solvent. Up until this point, we’ve been using a deuterated solvent (D2O, or “d-water”), where hydrogen atoms are replaced with deuterium atoms, to study the type I process. Researchers regularly use this solvent because singlet oxygen—which has a very weak emission signal—has a longer lifetime in it and thus is easier to monitor. However, you wouldn’t put a deuterated solvent in the human body; you would use regular water. Monitoring singlet oxygen in regular water requires a powerful laser and a highly sensitive detector.
You mentioned that your students also conduct research at the CFN. What role does hands-on research play in science, technology, engineering, and mathematics (STEM) education and how can user facilities like CFN help prepare students for future careers in STEM fields?
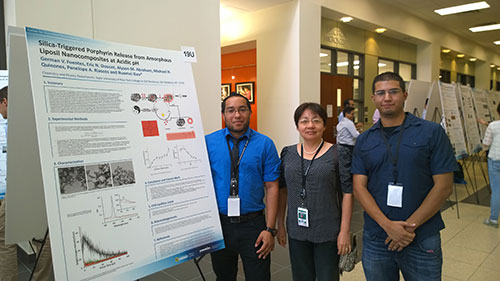
Gao with undergraduate students Eric Doucet (left) and German Fuentes (right), who presented their research on the pH-triggered release of porphyrin for photodynamic therapy during the Department of Energy Triennial Review at the CFN on August 23, 2016. On April 8, 2017, Fuentes won first place in the New York State Collegiate Science and Technology Entry Program (CSTEP) Chemistry I Poster Research Competition and the Dr. Henry Teoh Award for Outstanding Research Poster.
Since joining Old Westbury, I’ve been actively working with undergraduates in the research laboratory. Bringing research into the classroom is an important part of my teaching philosophy. From 2016 to 2020, I was the principal investigator (with co-principal investigators Judith Lloyd, Bright Emenke, and Duncan Quarless) of a National Science Foundation Improving Undergraduate STEM Education (NSF-IUSE) project. My colleagues and I used two teaching sequences to develop students’ argumentative abilities: a writing workshop divided into four modules for conceptual learning and weekly laboratory report writing for knowledge application. The four writing workshop modules guided students through the processes of identifying key components of an argument (evidence, justifications, and claims), selecting appropriate and inappropriate justifications, constructing strong justifications and conclusions, and analyzing experimental errors. We encouraged students to design their own experiments and engage in argumentative writing in chemistry laboratories.
Two of my former students, Eric Doucet and German Fuentes, did some of their research lab work at the CFN. Their time at the CFN was unlike any other experience they’ve ever had in college. Engaging in hands-on research at the CFN gave them a sense of what science is and how scientists work. The research results they got at the CFN were published and made them feel their work had real impact; it wasn’t just for the grade or degree. Several of my students have presented their research at annual Collegiate Science and Technology Entry Program (CSTEP) statewide conferences as well as other undergraduate research conferences, and some of them have won prizes. These achievements greatly benefited from the support of CFN staff and facilities.
How did you become interested in analytical chemistry and photochemistry?
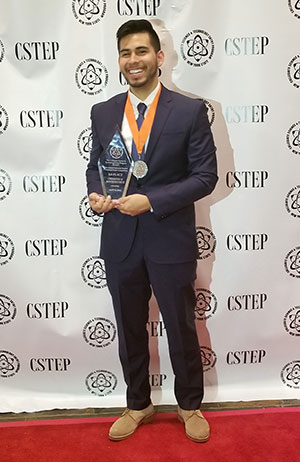
On April 14, 2018, SUNY Old Westbury undergraduate Nelson Euceda, mentored by Gao, took first place in the Chemistry/Biochemistry Poster Research Competition of the New York State Collegiate Science and Technology Entry Program (CSTEP) for the project, "UVA-Induced 6-Thioguanine Superoxide Radical Production Enhanced by Gluthathione."
My foundational interest in analytical chemistry began during my master’s studies. I started with inorganic analysis and then moved to electroanalytical chemistry, studying compounds that show activity when you apply an electrical potential.
My first experience in photochemistry was as a postdoctoral fellow with Joseph Rabani at Hebrew University of Jerusalem in Israel. There, I applied pulse radiolysis, a method for rapidly generating reduced or oxidized chemical species and free radicals. Then, I studied singlet oxygen chemistry in Matthias Selke’s laboratory at California State University at Los Angeles. So, both of my postdocs were related to photochemistry. I consider myself an analytical chemist with a strong expertise in photochemistry.
I find this field very interesting and exciting. It involves not only doing experiments but also theoretical work to understand the data you collect. It’s easy to explain to students. Everyone already knows what light and oxygen are, so I only need to teach them what photosensitizers are and how they work. In the future, I hope to provide students with more opportunities to do research in my laboratory, where we perform basic research to understand the mechanisms of the primary processes for producing reactive oxygen species. If we can make these mechanisms clear, it will help the applications—improving the selectivity of photodynamic therapy toward cancer cells and reducing side effects of cancer drugs.
Interested in becoming a CFN user? Submit a proposal through the CFN Proposal Portal. The next deadline is May 31. If you have questions about using CFN facilities or partnering with CFN scientists, please contact CFN Assistant Director for Strategic Partnerships Priscilla Antunez at (631) 344-6186 or pantunez@bnl.gov.
Brookhaven National Laboratory is supported by the U.S. Department of Energy’s Office of Science. The Office of Science is the single largest supporter of basic research in the physical sciences in the United States and is working to address some of the most pressing challenges of our time. For more information, visit https://energy.gov/science.
Follow @BrookhavenLab on Twitter or find us on Facebook.
2021-18907 | INT/EXT | Newsroom