Making High-Quality Materials with Mingzhao Liu
interview with a CFN staff member
September 15, 2021
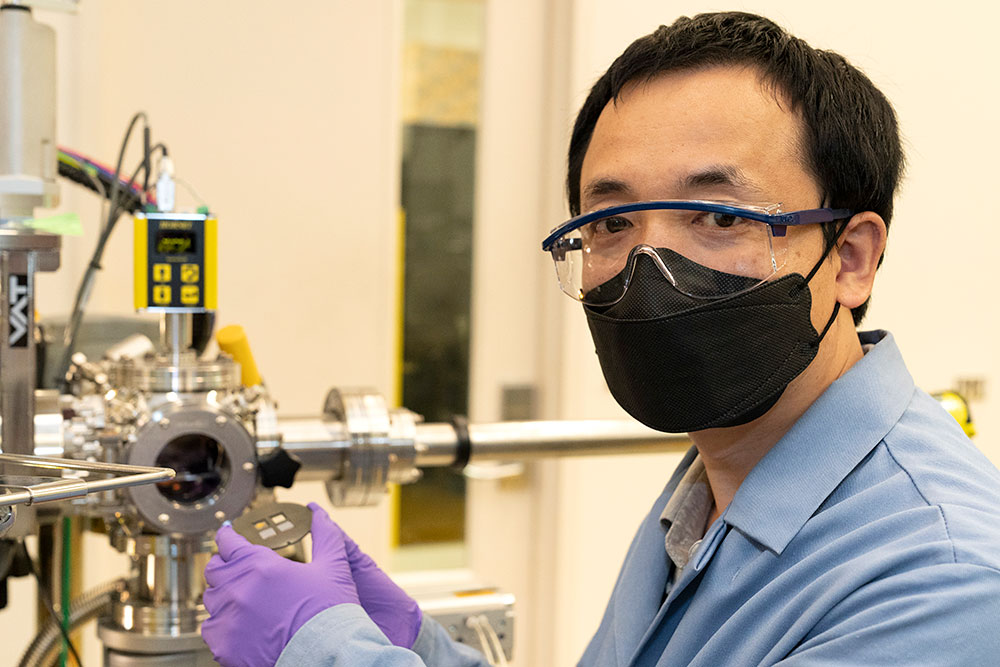
Mingzhao Liu holds a substrate holder in front of the pulsed laser deposition system in the Materials Synthesis and Characterization Facility at the Center for Functional Nanomaterials (CFN) at Brookhaven National Laboratory. Liu manages this system for CFN staff and users and applies it to his own research to precisely grow complex, highly crystalline materials like metal oxides.
How can we make better materials—those that are more energy efficient and functionally capable? This question is one that Mingzhao Liu has been thinking about as he fabricates thin-film materials for applications in solar energy conversion and quantum information science (QIS). Liu joined the Center for Functional Nanomaterials (CFN)—a U.S. Department of Energy (DOE) Office of Science User Facility at Brookhaven National Laboratory—in 2012 as a staff scientist in the Electronic Nanomaterials Group and moved over to the Interface Science and Catalysis Group in 2016. Recently, he was appointed a materials subthrust leader of the Brookhaven Lab–led Co-design Center for Quantum Advantage (C2QA), which is building the fundamental tools necessary to create scalable, distributed, and fault-tolerant quantum computers. Since 2014, Liu has also served as an adjunct professor in the Department of Materials Science and Chemical Engineering at Stony Brook University, helping to train the next generation of scientists. He completed a postdoctoral fellowship in the Department of Chemistry and Chemical Biology at Harvard University, after receiving his PhD from the University of Chicago and bachelor’s degree from Peking University, both in chemistry.
Solar energy conversion and QIS are two booming fields. How did you come to perform materials science research in both at the CFN?
My PhD research focused on plasmonics, which is all about light-matter interactions in metals, like the shine you see when a metal is struck by light. On the nanoscale, these interactions become far more interesting than on the bulk scale.
During my postdoc, I began exploring how interactions between light and metal nanoparticles could be used to promote solar energy conversion. I found solar chemistry very interesting and realized there were lots of problems scientists didn’t fully understand because they didn’t have “perfect” materials. To probe the true properties of materials, we need to grow them with as few defects—for example, domain boundaries (interfaces where two crystals with different orientations meet) and oxidized surfaces—as possible. When I joined the CFN, I shifted my research to focus entirely on finding materials that can absorb solar radiation with high efficiency and maintain chemical stability against harsh environments.
A newer interest within the DOE and now part of my research is QIS. Though QIS isn’t directly related to energy, in the sense we usually think of—collecting and storage energy—it indirectly is. Quantum computers could potentially perform computations much faster than conventional computers while using comparatively less power. But to make functional quantum devices, the QIS community also needs minimal-defect materials. These devices need to hold onto the quantum state for a long time to be practically useful, but materials always have defects that cause this state to decay.
Have you identified any promising materials for solar energy conversion?
Over the past few years, I’ve been developing a method based on pulsed laser deposition to precisely grow single-crystalline thin films of bismuth vanadate. Because it strongly absorbs sunlight across a range of wavelengths and remains relatively stable in water, bismuth vanadate is a promising electrode material for solar water splitting. In solar water splitting, solar energy is converted into chemical energy in the form of hydrogen, which can be stored for later use as a clean fuel.
My method involves striking the surface of a polycrystalline bismuth vanadate target with high-energy laser pulses. The heat from these pulses causes the atoms in the polycrystalline sample to evaporate and land on a substrate surface, forming a thin film.
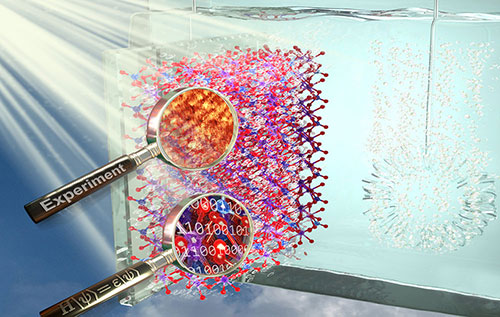
Through experiment and theory, Liu and colleagues showed how changes in the surface composition of the bismuth vanadate electrode play a critical role in photoelectrochemical performance. Research published in Nature Energy 6, 287 (2021).
In a study published in Nature Energy earlier this year, colleagues and I discovered that changing the composition of the top surface layer can have a dramatic impact on the catalytic activity of solar water splitting. When more bismuth exists on the surface than vanadium, the amount of electrical current generated from the absorption of sunlight is 50-percent higher!
Since this study, we’ve been using a lab-based ambient-pressure x-ray photoelectron spectroscopy (AP-XPS) instrument at the CFN to understand exactly what is happening chemically on the surface when water is introduced. Most tools do not have the sensitivity to probe the chemistry at the interface between a semiconductor and water. X-rays don’t travel very far in water, so that’s why the ambient pressure is important. We can condense some water on the surface to mimic the semiconductor-water interface while controlling the pressure to allow x-rays to pass through the system to do XPS. In this way, we can determine changes in surface composition, chemical state, and electronic structure when light and water exist together on the surface. Breakthroughs in solar water splitting will come from a true understanding of this surface chemistry.
You mentioned that QIS also faces a materials problem. Which materials have you been growing for QIS applications?
In the quantum community, discussions are still ongoing as to which kind of platform would be best suited for building quantum computing architectures. Most electronics today are based on silicon, an abundant and inexpensive material. Several companies are hoping to modify conventional silicon transistor technology for the quantum revolution. However, doing so would be difficult. A lot of superconducting metals—which could act as perfect conductors for quantum information processing—would form silicides (silicon combined with a more electropositive element) on silicon surfaces that are not superconducting.
Most research groups have been working with conventional metal-based materials, like aluminum, niobium, and, more recently, tantalum. But, in collaboration with colleagues from the CFN, Brookhaven’s Condensed Matter Physics and Materials Science Division, and Stony Brook University, I’ve been trying to grow superconducting metal silicides. We use a substrate called a silicon-on-insulator wafer, which contains a thick silicon base covered first by a thin silicon dioxide insulator and then by a thin crystalline silicon layer (usually called the device layer). We deposit a metal on top of this silicon device layer. When the metal and silicon react, a superconducting surface of metal silicide is formed. Then, we can start to think how to pattern this surface to make a device capable of hosting qubits, or quantum information bits. As we reported in Scientific Reports earlier this year, we synthesized thin films of the superconducting phase of vanadium silicide (V3Si), which has a relatively high superconducting transition temperature of 17 Kelvin (−429 degrees Fahrenheit). Now, our goal is to convert these superconducting vanadium silicide films into a working device.
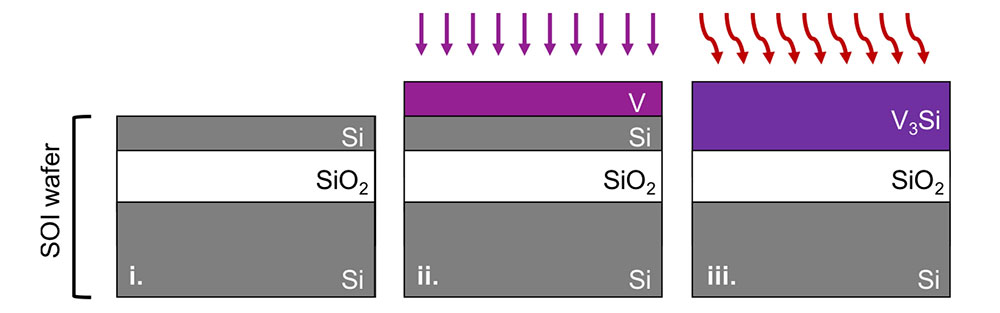
A schematic of the thin-film synthesis of vanadium silicide by reacting only a thin silicon device layer of a silicon-on-insulator (SOI) wafer. This device layer is completely converted into vanadium silicide without reacting with the underlying silicon dioxide. Vanadium is deposited by heat, causing it to evaporate (ii), and silicon is deposited by heating under vacuum. Research published in Scientific Reports 11, 2358 (2021).
In August 2020, the DOE selected Brookhaven Lab to lead one of five National QIS Research Centers: the Co-design Center for Quantum Advantage, or C2QA. The center targets three research thrusts: materials, devices, and software and algorithms. How did you become a materials subthrust leader, and what is your role?
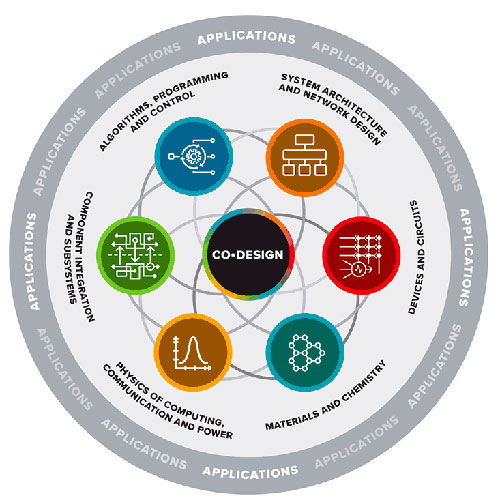
Co-design refers to the joint design of hardware and software. The Co-design Center for Quantum Advantage is developing and applying quantum co-design principles to target three research thrusts: software and algorithms, devices, and materials.
My training is as a materials scientist. When C2QA was first organized, me and other CFN staff had already been interacting with some of the C2QA partner institutions, including Yale and Princeton, on QIS research. When we started talking about the materials problems they were facing, we realized there were areas where CFN could contribute its expertise. For example, they were having difficulty making tantalum thin films. Tantalum has two different phases, with one phase becoming superconducting at a much lower temperature than the other, making it less useful for QIS devices. By finding the right conditions—such as temperature, background pressure, and deposition rate—I was able to grow high-quality thin films of tantalum containing only the phase with the higher superconducting transition temperature.
From this interaction, it became apparent how CFN could help support this new center. But the materials subthrust I’m leading goes beyond growing thin films. On a bigger picture, my role is to connect the team to the materials synthesis and characterization capabilities available at user facilities, including the CFN and National Synchrotron Light Source II (NSLS-II), also located at Brookhaven. Once we grow a material, we need to understand its properties and correlate these properties with device performance. Ultimately, we want to know, which material properties will lead to the best devices? Qubits are highly prone to errors because of defects in the qubit materials themselves and interactions with their environment. Through a feedback loop involving theory, materials synthesis and characterization, and quantum device measurements, we will uncover why these errors are occurring and tune the materials accordingly.
Which instruments at the CFN do you use to fabricate high-quality thin films?
The CFN has a large selection of tools to synthesize high-quality materials. The pulsed laser deposition tool I mentioned is best suited for growing complex materials—such as metal oxides and ceramics—with large-area, highly crystalline domains.
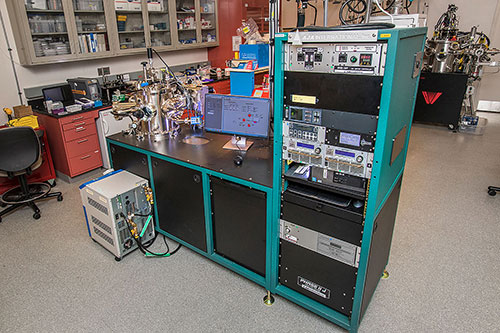
Liu is using this sputtering system, available in the CFN Materials Synthesis and Characterization Facility, to fabricate superconducting thin films for QIS research.
Another tool I use is for atomic layer deposition, a vapor-phase technique. Atomic layer deposition is ideal for achieving uniform and conformal (conforming to every place) coatings, not only on very smooth but also rough surfaces, such as those with pores. Scientists within the catalysis community often use this technique to grow catalytic thin films on substrates.
One of the key tools I’m now using for my QIS research on superconducting thin films is a sputtering system. It’s great for depositing metals. In my case, I bombard metal surfaces with accelerated ions of argon gas. The metal atoms get kicked out from the surface and land onto a heated substrate (such as a silicon wafer) to form a thin film. In the meantime, argon ions are flowing to prevent oxidation of the metal film. If the metal combines with oxygen, then the metal is no longer “perfect.” Ideally, to make quantum devices, we need to minimize domain boundaries and other defects because they act as scattering centers for the Cooper pairs of electrons that enable superconductivity. Disrupting the quantum coherence between these electron pairs reduces the length of time the quantum state can survive to perform computations.
After synthesizing the thin films, you need to understand their properties. Which characterization tools do you use at the CFN to study these properties?
I work a lot with CFN colleagues to characterize the thin films. For example, members of the CFN Electron Microscopy Group are experts in transmission electron microscopy (TEM), a useful technique for performing quality assurance checks. From TEM images, I can ask and answer questions such as how crystalline is the thin film? What is the nanoscale domain structure? What is the growth orientation? With help from colleagues in the CFN Interface Science and Catalysis Group, I can track the surface morphology and chemistry of the thin films, using tools like AP-XPS. I also frequently interface with the CFN Soft and Bio Nanomaterials Group, which manages several x-diffraction tools for structural characterization. These tools enable me to determine the material’s bulk crystalline structure. More recently, for the QIS research to turn the thin films into quantum devices, I’ve been leveraging instruments in the CFN Nanofabrication Facility, which houses a cleanroom.
When did you become interested in science and chemistry in particular?
I wanted to be a scientist my whole life. As a kid, I wanted to find out how things work and how to make them better. My father, an engineer, would bring home different kinds of chemicals for me to play with. I vividly remember him bringing home liquid glass one day. I was fascinated to see how this sticky liquid, when exposed to air, solidifies into a glass-like state.
Chemistry has always been one of my favorite subjects because you can see signs of change. More than that, you can rationalize why these changes occurred and use that to solve problems. This problem-solving aspect drew me in during my childhood and throughout my education, and it continues to drive me today in my career. But now, instead of working on questions with known answers, as I did in high school, I’m trying to answer open questions for which we don’t yet have answers.
Brookhaven National Laboratory is supported by the U.S. Department of Energy’s Office of Science. The Office of Science is the single largest supporter of basic research in the physical sciences in the United States and is working to address some of the most pressing challenges of our time. For more information, visit https://energy.gov/science.
Follow @BrookhavenLab on Twitter or find us on Facebook.
2021-19133 | INT/EXT | Newsroom